- 1Luiz de Queiroz College of Agriculture, University of São Paulo, Piracicaba, Brazil
- 2Centre for Environmental Sciences, Hasselt University, Diepenbeek, Belgium
- 3Department of Plant Physiology and Biophysics, Maria Curie-Skłodowska University, Lublin, Poland
- 4Centre for Mined Land Rehabilitation, Sustainable Minerals Institute, The University of Queensland, Brisbane, QLD, Australia
- 5School of BioSciences, The University of Melbourne, Parkville, VIC, Australia
- 6Laboratoire Sols et Environnement, Université de Lorraine – INRAE, Nancy, France
The pollution of soil, water, and air by potentially toxic trace elements poses risks to environmental and human health. For this reason, many chemical, physical, and biological processes of remediation have been developed to reduce the (available) trace element concentrations in the environment. Among those technologies, phytoremediation is an environmentally friendly in situ and cost-effective approach to remediate sites with low-to-moderate pollution with trace elements. However, not all species have the potential to be used for phytoremediation of trace element-polluted sites due to their morpho-physiological characteristics and low tolerance to toxicity induced by the trace elements. Grasses are prospective candidates due to their high biomass yields, fast growth, adaptations to infertile soils, and successive shoot regrowth after harvest. A large number of studies evaluating the processes related to the uptake, transport, accumulation, and toxicity of trace elements in grasses assessed for phytoremediation have been conducted. The aim of this review is (i) to synthesize the available information on the mechanisms involved in uptake, transport, accumulation, toxicity, and tolerance to trace elements in grasses; (ii) to identify suitable grasses for trace element phytoextraction, phytostabilization, and phytofiltration; (iii) to describe the main strategies used to improve trace element phytoremediation efficiency by grasses; and (iv) to point out the advantages, disadvantages, and perspectives for the use of grasses for phytoremediation of trace element-polluted soils.
Introduction
The concentrations of potentially toxic trace elements in the environment have been increasing year by year due to anthropogenic activities such as mining, smelting, untreated sewage disposal, and use of pesticides and fertilizers in agriculture (Kabata-Pendias, 2011). The widespread pollution of soil, water, and air with trace elements represent one of the most severe environmental problems that can seriously affect both environmental quality and human health (Food and Agriculture Organization of the United Nations [FAO] and United Nations Environment Programme [UNEP], 2021). For this reason, many civil engineering remediation processes such as chemical extraction, ion exchange, membrane separation, and electrokinetics were developed to reduce trace element contamination in the soil (Selvi et al., 2019). Despite the achievements of these processes, they face certain disadvantages including high cost, soil disturbance, and degradation, and also potentially lead to pollution of groundwater (Selvi et al., 2019). Plant-based technologies, generally termed phytoremediation, that are considered environmentally friendly, non-invasive, energy-efficient (mainly sun-powered), and cost-effective to remediate sites with low-to-moderate concentrations of trace elements are proposed as alternative approaches (Vangronsveld et al., 2009). Nevertheless, if phytoremediation is chosen to remediate trace element pollution, the question is which kind of plant is the most suitable for this purpose? No general answer to this question can be given, as there exist different options for different cases (Tordoff et al., 2000; Vangronsveld et al., 2009).
Grasses used for biofuel or fiber production and as well as grasses normally used for animal feed (in this case the forage produced cannot be used for feedstock for animals) are potentially suitable candidates for phytostabilization, and in some cases phytoextraction, since most species have extensive root systems (which allows for the exploration of large soil volumes), high biomass yields, fast growth rates, adaptations to soil infertility, and successive shoot regrowth after harvest (Rabêlo et al., 2018a). The first studies assessing the ability of grasses to persist in environments polluted by trace elements were conducted in the early 1950s, while research in this field was boosted from the 1990s onward (Figure 1). Although most grasses have desirable characteristics for trace element phytoremediation, some genera have been more extensively studied than others (Figure 2). The greatest number of studies have been undertaken with the grasses of the genera Phragmites, Lolium, Sorghum, and Festuca are because of their large geographical distribution globally, extending from cold regions to humid wetlands in the tropics (Zeven and de Wet, 1982), and their higher tolerance to trace elements exposure. However, the potential of each grass species for trace element phytoremediation depends on several factors such as trace element bioavailability (Rabêlo et al., 2020a), the mechanisms involved in the uptake, transport, accumulation, toxicity, tolerance to each trace element (Sipos et al., 2013), and the cultivation system (Tordoff et al., 2000; Vymazal and Březinová, 2016), among other factors.
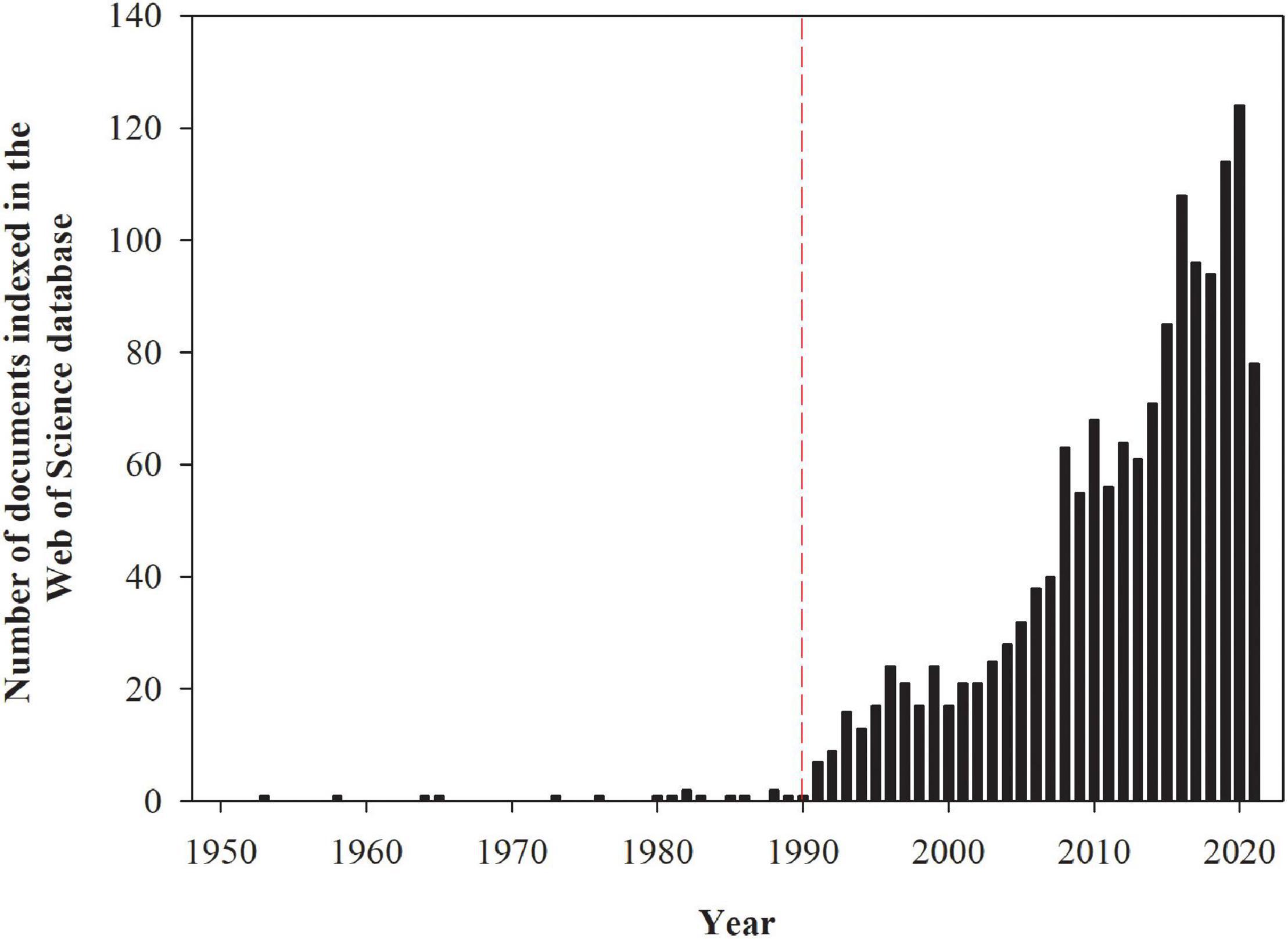
Figure 1. The number of documents indexed in the Web of Science database assessing the ability of grasses to survive in environments polluted by potentially toxic trace elements. The numbers presented in the figure were obtained from the search with the keywords grass and heavy metals on August 4, 2021. These numbers are subjected to changes according to keywords used for search.
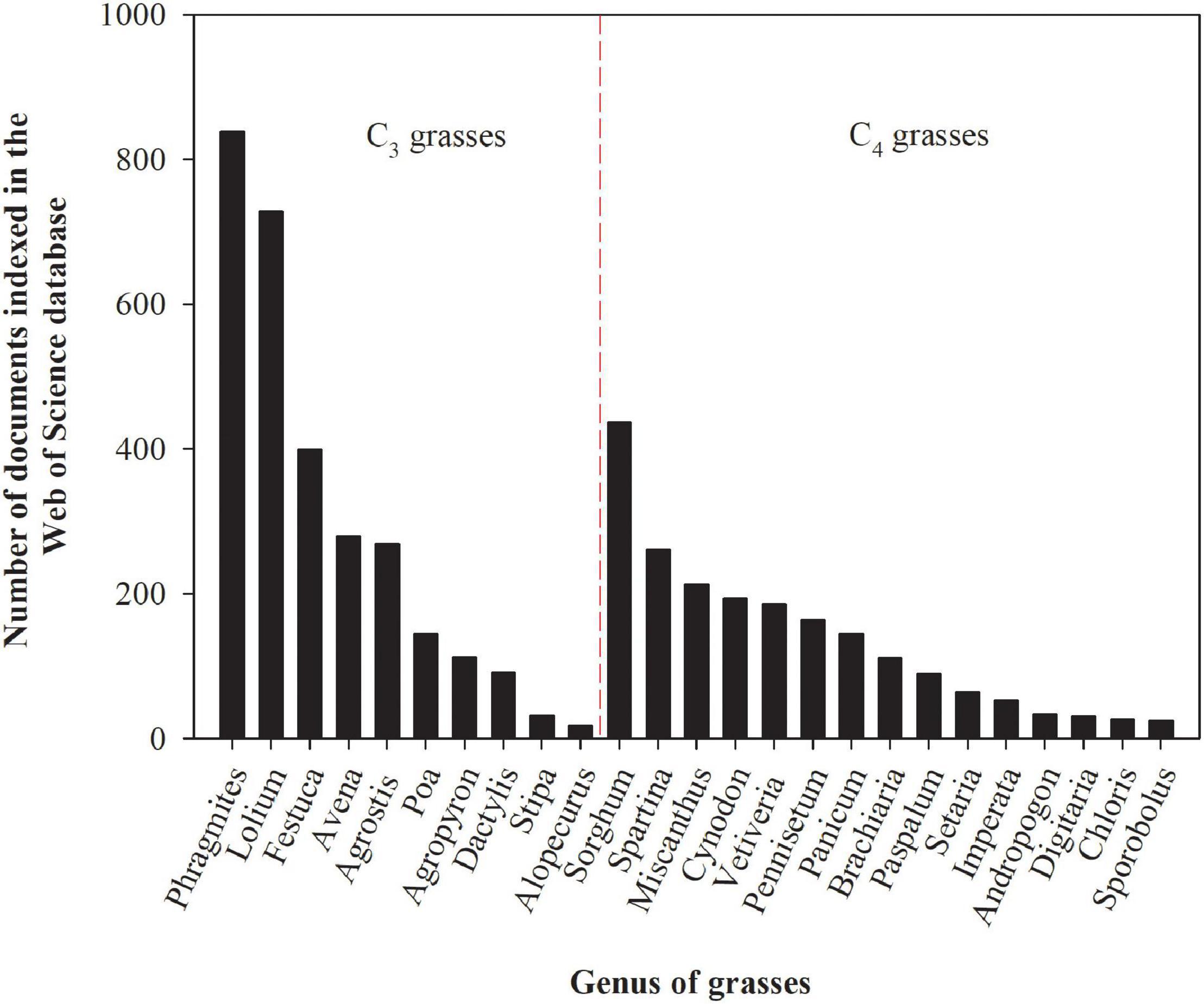
Figure 2. The number of documents indexed in the Web of Science database assessing the tolerance and capacity of grasses genera (C3 and C4) to remediate environments polluted by potentially toxic trace elements. The numbers presented in the figure were obtained by using the name of the genus and the keyword heavy metals as search terms on February 22, 2021. These numbers are subjected to changes according to keywords used for search.
This review aims to (i) to synthesize the available information concerning the mechanisms involved in the uptake, transport, accumulation, toxicity, and tolerance to trace elements in grasses; (ii) to identify suitable grasses for phytoextraction, phytostabilization, and phytofiltration; (iii) to describe the main strategies used to improve the phytoremediation efficiency by grasses; and (iv) to point out advantages, disadvantages, and perspectives for the use of grasses for phytoremediation of polluted soils.
Uptake, Transport, Accumulation, and Toxicity of Trace Elements in Grasses
In the context of environmental pollution, the most important trace elements are arsenic (As), cadmium (Cd), chromium (Cr), copper (Cu), mercury (Hg), nickel (Ni), lead (Pb), and zinc (Zn) (He et al., 2015). These trace elements can be subdivided into essential (Cu, Ni, and Zn) and non-essential trace elements (As, Cd, Cr, Hg, and Pb) for plants, but both essential and non-essential trace elements can be toxic, even at low prevailing concentrations (Clemens, 2006). For this reason, many studies evaluating uptake, transport, accumulation, and toxicity of trace elements in grasses for phytoremediation applications have been conducted (Figure 3). We summarize below the main findings related to these processes for As, Cd, Cu, Hg, Ni, and Zn in grasses. Chromium and Pb are not covered in this section since both are highly insoluble (and hence unavailable for uptake) under natural conditions (Senila, 2014; Ertani et al., 2017), and most of the studies conducted with grasses used unrealistically high Cr and Pb dose rates in hydroponic solution, leading to spurious results.
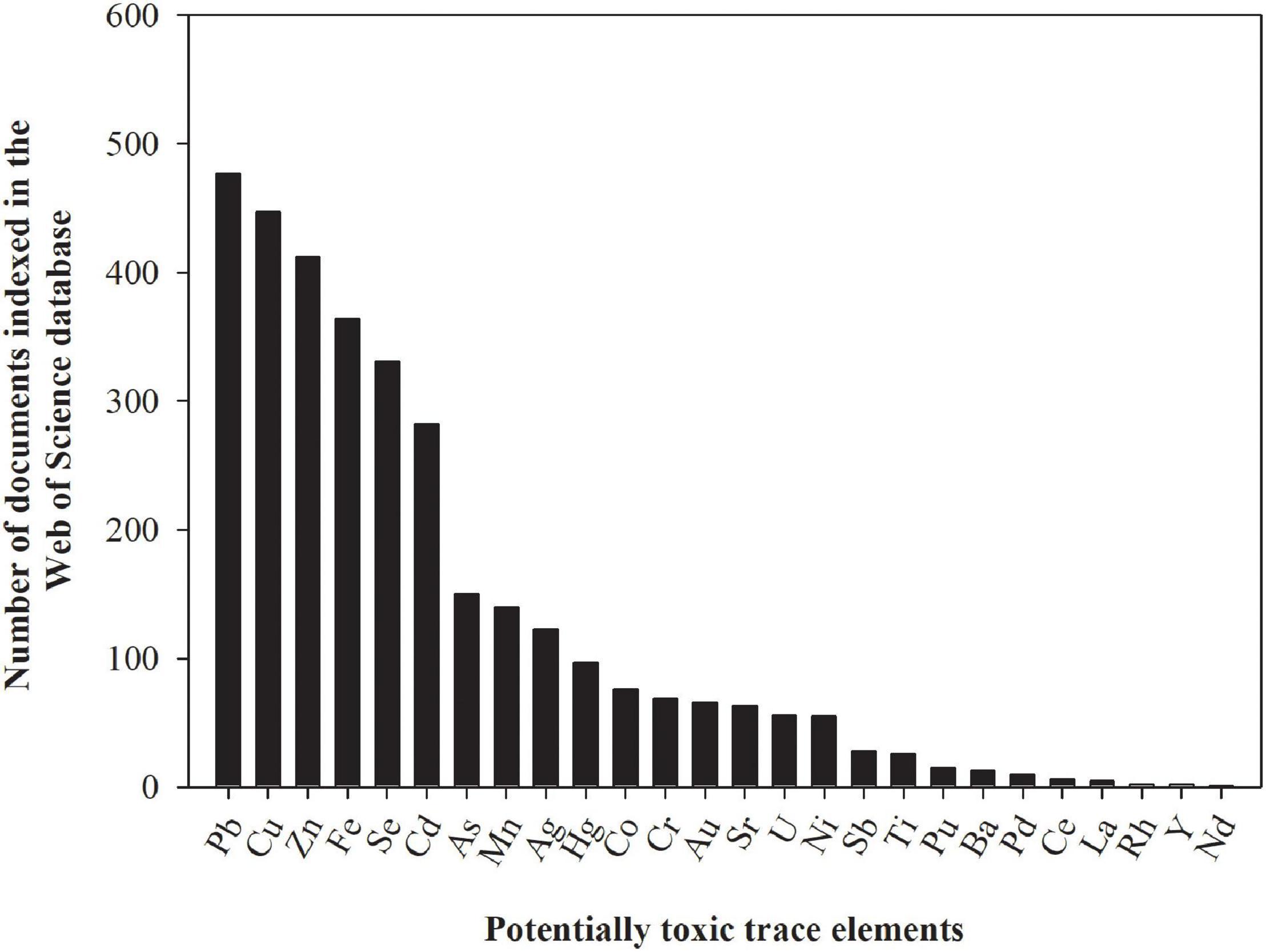
Figure 3. The number of documents indexed in the Web of Science database assessing the effect of potentially toxic trace elements on tolerance, survival, and/or remediation capacity of grasses. The numbers presented in the figure were obtained by using the name of the potentially toxic trace element and the keyword grass as search terms on February 23, 2021. These numbers are subjected to changes according to keywords used for search.
Arsenic
Non-essential elements move into and are transported through a plant along the same routes as essential elements (Clemens and Ma, 2016). Arsenic can be taken up by plants as arsenate (AsO43–) from aerobic soil through phosphate transporters, and as arsenite (AsO33–) from flooded soil through silicon (Si) transporters (Ma et al., 2008). Molecular studies identifying transporters involved in As uptake in monocots are scarce (except for commercial crops such as rice). To examine the contribution of cationic transporters for anions uptake, the P1B-ATPase genes family was implicated (Darabi et al., 2017). The expression of HMA2, HMA5, and HMA7 increased in the roots of Sorghum bicolor in response to As exposure, suggesting that many putative pathways exist between As and Cd and P1B-ATPases to stimulate the expression or inhibition of this family. Arsenic toxicity may alter some cationic trace element transporters and induce a level of toxicity to produce synergy or may result in incompatibility between transporters when a plant is exposed to a trace element. However, it is well documented that heavy metal ATPases (HMA) transporters are involved in both Cd and Zn translocation from roots to shoots (Wong and Cobbett, 2009). After uptake, As predominantly accumulates in the plant tissues as AsO33–, which is also the main form of As translocated from the roots to shoot (Peralta-Videa et al., 2009). The reduction of AsO43– to AsO33– is catalyzed by the enzyme arsenate reductase (AR) (EC 1.20.99.1) that is encoded by the arsC gene (Zhao et al., 2009). Vetiveria zizanioides (also known as Chrysopogon zizanioides) accumulated As in its shoot at a low level, which was attributed to As-induced toxicity due to the absence of both transcripts of arsC and AR activity (Tiwari et al., 2015). In fact, plants presenting low AR activity tend to accumulate more As in their shoots than plants with high AR activity (Dhankher et al., 2002). A rapid reduction of AsO43– to AsO33– catalyzed by AR often is followed by complexation with thiols and possibly sequestration into the root vacuoles, which results in lower mobility and translocation of As from the roots to the shoot, except in hyperaccumulators (Zhao et al., 2009). Possibly, due to these As detoxification processes, other grass species, such as Agrostis capillaris, Holcus lanatus (Dradach et al., 2020b), Festuca rubra (Dradach et al., 2020a), and Pennisetum purpureum (also known as Cenchrus purpureus) (Kowitwiwat and Sampanpanish, 2020), have higher As concentrations in the roots than in the shoots.
Symptoms of As-induced toxicity are closely related to changes in the integrity of plasma membranes that affect nutrient uptake and plant water status. Arsenic interacts with functional groups of enzymes, replaces phosphate groups from the ATP, and suppresses key events related to the activity of photosystem II (PSII) (for a comprehensive review see Abbas et al., 2018). An excess of As leads to the production of reactive oxygen species (ROS) in the cell which can disturb the redox balance and cause oxidative stress. The generation of ROS in plants exposed to As should be linked to the reduction of AsO43– to AsO33– (Abbas et al., 2018). The exposure to high As concentrations (10, 50, 100, and 200 μmol L–1) induced oxidative stress in both the roots and shoots of V. zizanioides after 7 and 14 days of exposure (Singh et al., 2017). Nevertheless, the low or absent AR activity in V. zizanioides (Tiwari et al., 2015) suggests that ROS generation in grasses can also occur from other As-induced biochemical changes. Arsenic negatively affects seed germination. Diminished seed germination in F. rubra exposed to As concentrations higher than 6 mg L–1 was reported (Vázquez de Aldana et al., 2014). Other studies describing symptoms of As-induced toxicity in grasses are presented in Table 1.
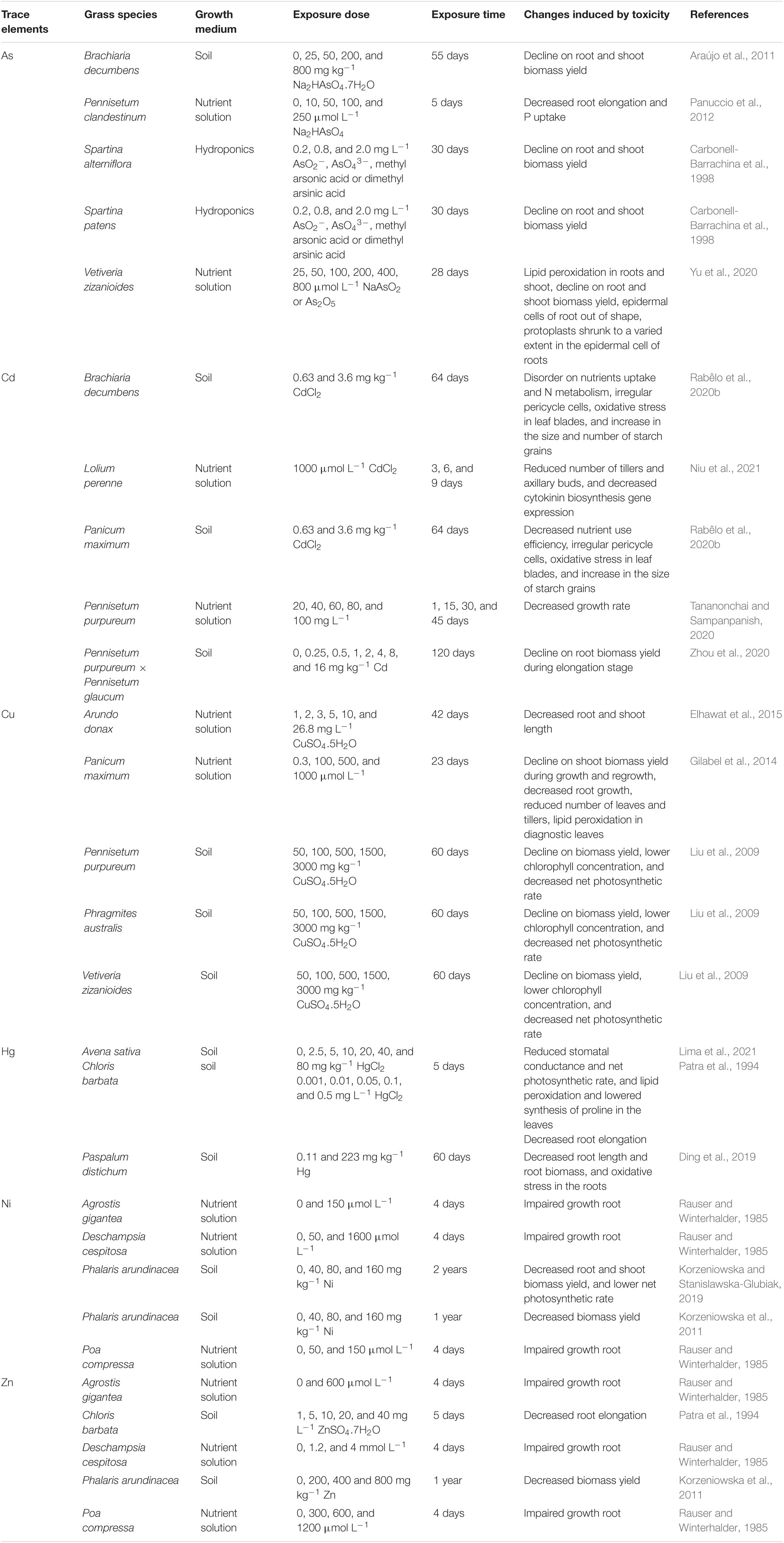
Table 1. Symptoms of toxicity induced by As, Cd, Cu, Ni, or Zn in grasses assessed for phytoremediation.
Cadmium
Unlike the anions AsO43– and AsO33–, cations such as Cd2+ can reach the vascular system of plants through the apoplastic and symplastic pathways (Lux et al., 2011). In an attempt to evaluate the contribution of apoplastic and symplastic pathways for Cd uptake in Panicum maximum (synonym Megathyrsus maximus), Rabêlo et al. (2017c) reported that Cd was taken up through both pathways, but that the symplastic contribution was affected by sulfur (S) supply, probably due to S-induced changes in the synthesis of phytochelatins (PCs) [(γ-Glu-Cys)n-Gly, with n = 2–11]. These S-rich compounds are involved in Cd2+ sequestration from the cytosol to the vacuole (Rabêlo et al., 2018b). From the symplastic pathway, Cd can enter root cells as Cd2+ through Zinc-regulated transporter/Iron-regulated transporter-like Protein (ZIP) transporters and Cd-chelates through Yellow-Stripe 1-Like (YSL) proteins, whereas transporters of the P1B-ATPases family are involved in Cd loading into the xylem (Lux et al., 2011). The genes HMA5 and YSL5 were upregulated in response to Cd, and both contributed to Cd transport from the symplast into the xylem in P. americanum × purpureum plants (Zhao J. et al., 2019). In F. arundinacea (also known as Schedonorus arundinaceus), the HMA3 gene was found to play a key role in the Cd translocation from the roots to the shoots (Zhu et al., 2020). On the other hand, several transporters of the P1B-ATPases family were downregulated in the roots and shoot of S. bicolor exposed to 50 μmol Cd L–1, but HMA8 was up-regulated at a Cd concentration of 500 μmol Cd L–1 in solution, leading to the conclusion that this gene has a role in the adaptation of sorghum to Cd (Zhiguo et al., 2018). These results indicate that the expression of genes related to Cd transport is tissue- and species-specific.
Cadmium concentrations in the roots and shoots are mainly determined by the Cd entry into the roots, sequestration within root vacuoles, translocation in both xylem and phloem, and dilution within the shoot due to growth (Lux et al., 2011; Clemens and Ma, 2016). Among the metabolites involved in Cd sequestration into the vacuoles, the most important are PCs that exert a great influence on Cd accumulation, mainly in the roots (Clemens, 2006). Cadmium accumulation in the roots of P. maximum was closely related to PCs synthesis in plants exposed to 100 or 500 μmol Cd L–1 in solution for 9 days (Rabêlo et al., 2018b). However, in plants that are longer exposed to Cd, binding by PCs in the roots is decreasing. In the roots of Brachiaria decumbens (also known as Urochloa decumbens) and P. maximum grown for 64 days in an Oxisol with 3.6 mg Cd kg–1 (Rabêlo et al., 2021), the PC concentrations were only slightly higher than in the unpolluted control soil. Cadmium binding by cell walls (apoplast) was probably the process that most contributed to Cd accumulation in the roots of grasses under prolonged Cd exposure (Rabêlo et al., 2021). As speculated by Clemens (2006), Cd exposure time influences the binding partners for Cd accumulation. Even facing efficient mechanisms to avoid translocation from the roots to the shoot, Cd tends to accumulate more in the shoots compared with other trace elements (Clemens and Ma, 2016), in a process that is also influenced by transpiration (Lux et al., 2011). Nevertheless, comparing a potential Cd-accumulator (P. americanum × purpureum) and a Cd-excluder (V. zizanioides), the transpiration was correlated to the shoot Cd concentration only in the accumulator species (Zhang et al., 2014). The positive correlation between transpiration and shoot Cd accumulation is expected to exist only in tolerant grasses since Cd accumulation often decreases the leaf area of Poaceae plants, which is lowering the transpiratory flux (Kaznina and Titov, 2014).
Cadmium-induced toxicity symptoms in grasses may result from a wide range of interactions at the cellular level (Gallego et al., 2012). Cadmium exposure (15, 30, 60, and 100 mg Cd kg–1 soil) led to nutritional imbalance and reduced the net photosynthetic rate in P. americanum × purpureum and V. zizanioides (Zhang et al., 2014). Nutritional imbalance has been identified out as one of the main causes for growth inhibition and low biomass yield in two genotypes of P. maximum (Rabêlo et al., 2020b). Cadmium exposure (500–2,000 μmol L–1 in solution) also reduced the tillering in P. maximum, compromising the plant capacity to produce leaves and perform photosynthesis (Rabêlo et al., 2017a). Cadmium-induced changes of the photosynthetic apparatus have been suggested as the main cause for the low biomass yield in Cd-exposed Poaceae (Kaznina and Titov, 2014). Ultrastructural changes of the chloroplast and 60% lower ribulose 1,5-bisphosphate carboxylase/oxygenase (Rubisco, EC 4.1.1.39) activity in P. australis exposed to 100 μmol Cd L–1 in a solution were reported in comparison with non-exposed controls (Pietrini et al., 2003). Most studies to date involved acute Cd exposure (i.e., high exposure dose in a short time), however, from the perspective of phytoremediation, it is necessary to better understand the consequences of chronic exposure (i.e., low exposure dose for a long time).
Copper
Copper is taken up by transporters of the Copper Transporter (COPT) and ZIP families from the symplastic pathway, while transporters of the YSL and P1B-ATPases families are involved in Cu loading into the xylem (Andresen et al., 2018; Kumar et al., 2021). In Brachypodium distachyon, COPT3 and COPT4 are localized in the plasma membrane and are transcriptionally upregulated in both the roots and leaves by Cu deficiency, while COPT3, COPT4, and COPT5 confer low affinity to Cu transport (Jung et al., 2014). These results differ from those reported for other plants, in which COPT1, COPT2, and COPT6 were reported to have higher expression in the leaves, COPT3 and COPT5 are mainly expressed in the stems, and COPT4 in roots (Andresen et al., 2018). It is very probable, as also mentioned for Cd, that the expression of genes related to Cu transport is specific to tissues and grass species. Due to the strong binding of Cu2+ to the cell walls (Kopittke et al., 2011), the contribution of the apoplastic pathway to Cu uptake is believed to be low. Probably because of the action of the negative charges of the cell wall on Cu2+ binding, Cu is long-distance transported as Cu+ bound to metallothioneins (MTs) and/or complexed with organic ligands such as histidine, nicotianamine (NA), and 2’-deoxymugineic acid (Andresen et al., 2018). However, to the best of our knowledge, there exist no studies addressing the role of organic ligands in the long-distance transport of Cu in grasses used for Cu phytoremediation.
The limited number of Cu hyperaccumulator plants suggests that most plants are highly effective at excluding Cu from uptake and that this element is preferentially accumulated in the roots (Kumar et al., 2021). Panicum maximum exposed to Cu concentrations ranging from 0.3 to 500 μmol L–1 accumulated more Cu in the roots than the shoots, but the opposite was observed when this plant was exposed to 1,000 μmol L–1 Cu (Gilabel et al., 2014). Similarly, the Cu concentration in F. rubra exposed to 5 mg Cu L–1 was higher in the roots than in the shoots, but the opposite was found when this plant was exposed to 10 mg Cu L–1 (Malagoli et al., 2014). The Cu concentration in F. rubra and external Cu were dose-dependent (Malagoli et al., 2014), but most likely, Cu immobilization in the roots by cell wall binding, Cu chelation by PCs, MTs, and organic acids, or Cu compartmentation in the vacuoles are not sufficient in situations of extremely high Cu concentrations in the roots, and in this case, the plant translocates a great part of the Cu that is taken up to the shoot, which can lead to plant death. Copper distribution into the different aerial tissues in grasses is controlled by the nodes through a phloem-kickback mode (for a comprehensive review see Yamaji and Ma, 2014). In this sense, high Cu concentrations in the nodes could cause Cu toxicity and alter Cu distribution between the roots and the shoots. However, this assumption needs to be carefully investigated, because plants possess some mechanisms to cope with the excess of Cu, such as (i) immobilization of Cu in the roots (e.g., binding to cell walls) avoiding Cu translocation to shoot, (ii) stimulation of the efflux pumping trace element at the plasma membranes, (iii) chelation of Cu by PCs, MTs, organic acids, or heat shock proteins, and (iv) compartmentation of Cu in the vacuoles, which also affects the capacity of Cu accumulation (Kumar et al., 2021). For instance, a genotype of F. rubra containing higher concentrations of malic acid also contained higher Cu concentrations in its shoots Cu (Harrington et al., 1996).
At the cellular level, excess Cu can cause toxicity due to (i) binding to sulfhydryl groups in proteins, thereby inhibiting enzyme activity or protein function, (ii) induction of the deficiency of other essential ions, (iii) impaired cell transport processes, and (iv) oxidative damages (Andresen et al., 2018; Kumar et al., 2021). Souza Junior et al. (2019) evaluated the effects of 0.3, 250, 500, and 1,000 μmol Cu L–1 in the leaves and other shoot tissues of P. maximum and observed that the highest Cu concentrations induced oxidative stress in all tissues, but Cu exposure did not lead to increased hydrogen peroxide (H2O2) concentrations. This can be explained by the fact that transition trace elements like Cu catalyze the formation of hydroxyl radicals (⋅OH) from the non-enzymatic chemical reaction between superoxide (O2⋅–) and H2O2 through Fenton and Haber-Weiss reactions (Kumar et al., 2021). The Cu-induced generation of ⋅OH can strongly affect photosynthesis by decreasing the chlorophyll concentrations and affecting the PSII reaction centers (Richards et al., 2015; Kumar et al., 2021). Indeed, lower chlorophyll concentrations after Cu-exposure were reported for F. rubra (Malagoli et al., 2014), while the lower electron transport rate (ETR) and quantum yield of PSII (ϕPSII) were described in P. maximum (Souza Junior et al., 2019). These results indicate that the Cu-induced toxicity effects on photosynthesis are similar for C3 and C4 grasses. As a result of Cu-induced physiological changes, a decline of biomass production, reduction of root growth, chlorosis, bronzing, and necrosis are the most common symptoms associated with exposure to excess Cu (Kumar et al., 2021). Some of these Cu-toxicity symptoms are described in Table 1.
Mercury
Compared with other trace elements such as As and Cd, information about Hg uptake by plants is scarce (Clemens and Ma, 2016). It is believed that Hg is readily taken up by plant roots through a low-affinity root transporter and predominantly accumulated in the roots (Esteban et al., 2008; Chen and Yang, 2012). Mercury (Hg2+) import into root cells occurs possibly through Fe, Cu, or Zn transporters/channels (Chen and Yang, 2012). A kinetical study pointed out that Hg accumulation in the roots is dose-time-dependent and shows saturable behavior for the lower Hg concentrations and linear behavior for the highest ones (Esteban et al., 2008). The Hg2+-ion easily interacts with anionic compounds (e.g., sulfate and phosphate) forming insoluble precipitates, which limit its symplastic mobility (Chen and Yang, 2012). In V. zizanioides exposed to 0.8 mg L–1 HgCl2, Hg was mainly found in the root epidermis and exodermis (tissues containing high lignin), whereas trace levels of Hg were localized in the vascular bundles (Lomonte et al., 2014). Approximately 80% of the Hg trapped in the roots is bound to the cell walls (Wang and Greger, 2004). Therefore, Hg translocation from the roots to the shoots is believed to be very low (Chen and Yang, 2012; Lomonte et al., 2014). The shoot, particularly the leaf, is another important route for Hg0 uptake due to the industrial emissions of Hg to the air and microbial-mediated Hg emissions from soils (Chen and Yang, 2012). Indeed, the Hg concentration in the leaves of Lolium perenne increased linearly with the Hg concentrations in the air (De Temmerman et al., 2007). The mechanisms of how gaseous Hg enters leaves remain elusive, but the stomata may be responsible for the uptake of Hg by the leaves through gas exchange (Chen and Yang, 2012; Shahid et al., 2017). In plants exposed to Hg0 in the atmosphere, the Hg accumulated in the shoot does not move to the roots (Suszcynsky and Shann, 1995). This statement apparently is also applicable to grasses. Niu et al. (2013) measured the Hg concentrations in the roots and leaves of L. perenne exposed to gaseous Hg and/or Hg-containing soil and found that Hg originating from the soil had a significant influence on the Hg accumulation in the roots, but had no influence on the Hg accumulation in the leaves that were accumulated from the atmosphere. Niu et al. (2013) concluded that Hg presence in the leaves of grasses is mainly of atmospheric origin. In summary, Hg accumulation depends on if the plant is exposed to elemental (vapor) Hg0 through the shoot or Hg2+ ions through roots (Suszcynsky and Shann, 1995).
The toxicity due to Hg exposure is often associated with ROS generation, disturbance of almost any function in which critical or non-protected proteins are involved due to the high affinity of Hg for sulphydryl (–SH), negative effects on both light and dark reactions of photosynthesis, and inhibition of the water channels in the membrane of higher plant cells (Patra et al., 2004; Chen and Yang, 2012). The photosynthetic rate, stomatal conductance, and transpiration decreased in Avena sativa grown in an Oxisol (Typic Hapludox) polluted with 2.5–80 mg Hg kg–1 soil, but no oxidative stress in the leaves was observed (Lima et al., 2021). Possibly, the Hg concentrations in the leaves of A. sativa were not high enough to induce oxidative stress, or the Hg2+ was reduced inside the plant into Hg0 to avoid the binding of Hg2+ to –SH groups of antioxidative compounds. Both Phragmites australis and Spartina alterniflora can release Hg0 from their leaves and thus lower Hg toxicity, in a process dependent on Hg2+ reduction into Hg0 (Windham et al., 2001). As roots are the main site of Hg2+ accumulation, inhibitory effects on root growth are expected. The root elongation of Iseilema membranaceum, Dichanthium sericeum, and Sporobolus africanus decreased after 28 days of exposure to 5,000 mg Hg L–1 (Mahbub et al., 2017). More studies describing the symptoms of Hg-induced toxicity are presented in Table 1.
Nickel
Nickel is taken up by plant roots via passive diffusion and active transport, but the ratio of uptake between active and passive transport varies with the species, the chemical form of Ni, and its concentration in the growth medium (Yusuf et al., 2011). Chintani et al. (2021) studied the Ni uptake by V. zizanioides and found that the uptake rate and efflux rate of Ni were dose-time-dependent, but no mechanisms related to Ni uptake were elucidated. To date, there is no evidence for the existence of Ni-specific transporters in plants. Nickel uptake by roots appears to occur through poorly selective cation transporters, notably members of the ZIP family that are responsible for Fe and Zn uptake (van der Pas and Ingle, 2019). In line with this hypothesis, the inhibition of Ni accumulation by Zn and Fe has been reported in Ni hyperaccumulator plants (Taylor and Macnair, 2006). After being taken up, the chelation of Ni in the cytosol by ligands is thought to be an important process in the root-to-shoot Ni transport (Yusuf et al., 2011). The preferred, but not exclusive, ligand of Ni is histidine (Andresen et al., 2018). Nicotianamine also appears to be involved as a ligand in the long-distance transport of Ni (Andresen et al., 2018). Nickel accumulation in the shoot of L. perenne was closely related to high xylem transport rates and citric acid concentration (Yang et al., 1997), which suggests that this organic acid is also involved in Ni translocation in grasses. Although Ni can also enter plants via leaves (Yusuf et al., 2011; Shahid et al., 2017), the main routes of Ni uptake and transport in plants are from the roots to the shoots through the transpiration stream via the xylem (Yusuf et al., 2011). A positive correlation was observed between Ni accumulation in the shoot of Agropyron elongatum and transpiration (Sipos et al., 2013), which suggests that Ni translocation in this species is driven mainly by transpiration. However, other processes also influence Ni translocation from the roots to the shoot in plants, such as Ni chelation. In A. elongatum, the Ni translocation factor (TF) remained below 0.38, regardless of the Ni concentration in the soil (Chen and Wong, 2006). A low Ni accumulation in the shoot has been associated with the absence of Ni ligands since without being chelated by ligands, Ni translocation from the roots to the shoot is expected to be retarded as xylem cell walls have a high cation exchange capability (CEC) (Yusuf et al., 2011). On the other hand, histidine could promote the root-to-shoot translocation of Ni by reducing the vacuolar sequestration of Ni in the root tissues of hyperaccumulators (van der Pas and Ingle, 2019). The same probably occurs when citric acid is functioning as a Ni ligand (Yang et al., 1997). Thus, the higher Ni accumulation in the shoots than the roots in Cynodon dactylon and F. arundinacea (Soleimani et al., 2009) could be related to a higher synthesis of histidine and/or citric acid.
The toxicity induced by Ni is mainly related to (i) the displacement of essential constituents in biomolecules, (ii) binding to essential biological functional groups of the molecules, and (iii) modification of enzyme/proteins, plasma membrane, and/or membrane transporters structure/function, which can lead to nutritional imbalance (Yusuf et al., 2011). Nickel exposure decreased both manganese (Mn) and Zn concentrations in the shoots of L. perenne (Khalid and Tinsley, 1980). Also, a lowered Mn concentration was reported in the shoots of Ni-exposed Urochloa mosambicensis, B. decumbens, Chloris ventricose, Astrebla lappacea, and C. ciliaris, as well as lower biomass yield due to Ni toxicity (Kopittke et al., 2010). Nickel accumulation limited the biomass yield of P. maximum, B. brizantha, and B. decumbens (Silva et al., 2017). Furthermore, Ni can disturb the balance between the formation and scavenging of ROS in plant cells (van der Pas and Ingle, 2019), resulting in oxidative stress. Nickel also damages the photosynthetic apparatus by impairing mesophyll cells and epidermal tissues, reducing chlorophyll content, and increasing the number of non-appressed lamellae (Yusuf et al., 2011). Studies describing symptoms of Ni toxicity at the molecular level in grasses are very scarce. Most studies focus on the general symptoms of Ni-toxicity, such as root growth inhibition and decreased biomass yield (Table 1).
Zinc
Zinc is taken up from the soil solution as Zn2+ or complexed with organic ligands. The roots feed the shoot via the xylem (Broadley et al., 2007). Grasses acquire (extra?) Zn by releasing mugineic acid for ferric ion (Fe3+) uptake [Strategy II for iron (Fe) uptake]. This phytosiderophore chelates in addition to Fe3+ also Zn2+ (Andresen et al., 2018). Also, derivatives of mugineic acid such as 3-epi-hydroxymugineic acid are involved in Zn uptake by grasses grown in calcareous soils, as described by Cakmak et al. (1996) for A. orientale. The Fe3+-phytosiderophore transporter YS1 also transports mugineic acid complexed with Zn2+ (Schaaf et al., 2004). Zinc loading into the xylem is accomplished by active Zn export out of the symplast, mediated by members of the HMA of the P1B-ATPases family (Andresen et al., 2018). Besides the symplastic pathway, Zn can be delivered extracellularly to the stelar apoplast in zones where the Casparian bands are not fully formed (Broadley et al., 2007). In the xylem, Zn can be transported in its free form or bound to organic acids. How Zn is exported from the xylem into aerial tissues is not yet completely understood, but Zn translocation from the roots to shoots involves transporters of the ZIP family (Andresen et al., 2018). In Setaria italica and P. sumatrense, seven ZIP genes (ZIP1-ZIP7) are involved in Zn uptake, translocation, and storage (Alagarasan et al., 2017). This result is quite interesting if we consider that most of the Zn taken up by roots of grasses are preferentially distributed to the nodes and then delivered to developing tissues exclusively through the phloem, where the Zn loading into phloem is mediated by HMA2 transporters (Yamaji and Ma, 2014). In this sense, there is an important contribution not only of xylem but also phloem for Zn distribution in the shoots of grasses.
After being taken up, Zn can be accumulated in the roots in processes that depend on the synthesis of ligands, such as glutathione (GSH) (γ-Glu-Cys-Gly), MTs, PCs, or NA, to avoid uncontrolled interactions with binding sites or precipitation with anions, such as phosphate (Andresen et al., 2018). Consequently, a lower synthesis of Zn ligands leads to higher Zn accumulation in the roots. Zinc was much more accumulated in the roots than the shoots in Andropogon gayanus exposed to 142.3 up to 854.0 μmol Zn L–1 for 7 weeks (Ribeiro et al., 2020). Nardis et al. (2018) mentioned that in different genotypes of P. maximum, the Zn concentrations in the roots were higher than in the shoots. On the other hand, these authors also pointed out that in B. brizantha cv. Xaraes and B. decumbens, the Zn concentration was higher in the stem base (sometimes referred to as the basal node), followed by the shoot and roots, respectively. Commonly, the nodes of grasses contain concentrations of Zn that are more than ten times higher than those of other tissues at both the vegetative and reproductive stages. Grasses use the nodes as hubs for Zn distribution (Yamaji and Ma, 2014). Also, other factors influence Zn accumulation in grasses. Deng et al. (2006) investigated Zn accumulation in Beckmannia syzigachne, Leersia hexandra, Neyraudia reynaudiana, and Polypogon fugax, and found that the main tissue with the highest Zn concentration (roots or shoots) greatly varied in function of the Zn concentration in the growth medium, the plant species and the tolerance mechanisms employed to avoid Zn toxicity.
Zinc toxicity restricts both cell division and cell elongation in the roots, impairs cortical root cells, and inhibits Fe translocation (Rout and Das, 2003). Root elongation in B. syzigachne, L. hexandra, N. reynaudiana, and P. fugax decreased due to Zn excess (Deng et al., 2006). In P. maximum, B. brizantha, and B. decumbens, Zn toxicity also led to lower root growth (Nardis et al., 2018). In A. gayanus, Zn toxicity induced the vesiculation, vacuolation, and withdrawal of the plasma membrane from the root cell wall (Ribeiro et al., 2020). Zinc can also affect proteins, mainly the –SH groups of the plasma membrane and affects membrane stability. In addition, excess Zn can inhibit photosynthesis by affecting both photochemical processes and gas exchange (Rout and Das, 2003). Toxicity symptoms often become visible at leaf Zn concentrations higher than 300 mg kg–1 DW, but the toxicity thresholds can be highly variable even within the same species (Broadley et al., 2007). Lolium perenne contained more than 500 mg Zn kg–1 in its leaves when exposed to 1 mmol Zn L–1 or higher for 8 days, which decreased effective quantum yield of PSII (ϕPSII), maximal PSII photochemical efficiency (Fv/Fm), chlorophyll concentration, net photosynthetic rate, stomatal conductance, tillering and biomass yield (Monnet et al., 2005). Zinc can also change the normal functioning of the ascorbate-glutathione (AsA-GSH) cycle, affecting ROS scavenging in grasses (Rabêlo and Borgo, 2016). More symptoms of Zn-induced toxicity in grasses are presented in Table 1.
Tolerance Mechanisms of Grasses to Trace Element Toxicity
Trace element tolerance is not a simple physiological attribute, but a syndrome of adaptations at the cellular and biochemical levels (Baker, 1981). These adaptations appear to be involved primarily in avoiding the build-up of toxic concentrations at sensitive sites within the cells to prevent damaging effects (Hall, 2002). The strategies involved are diverse: (i) extracellularly they include the immobilization of trace elements by mycorrhizal associations, binding to cell walls, and chelation by root exudates; (ii) tolerance can also involve the plasma membrane, either by reducing the uptake of trace elements or by stimulating the efflux pumping of trace elements that have entered the cytosol; and (iii) within the cytosol, a variety of potential mechanisms exist, for example, for the repair of stress-damaged proteins involving heat shock proteins or MTs, and for the chelation of trace elements by organic acids, amino acids or peptides, or their compartmentation away from metabolic processes by transport into the vacuole (Hall, 2002; Kushwaha et al., 2016). When the employment of these tolerance mechanisms by plants is not sufficient to decrease the fraction of free trace elements within the cell, the antioxidative defense machinery is the next important ‘line of defense’ against trace element-induced toxicity (Gratão et al., 2005). Mechanisms that operate at the whole plant level, such as root-to-shoot transport, have an important role in trace element tolerance, but they are not covered in this section since they were briefly addressed above for each trace element. Specific tolerance mechanisms such as the release of Hg0 through the leaves to reduce Hg toxicity (Windham et al., 2001) were also not covered in this section.
The Role of Mycorrhizas in (Limiting) Trace Element Uptake
Mycorrhizal associations provide an effective exclusion barrier against trace element uptake by adopting absorption, adsorption, or chelation mechanisms that restrict the entry of trace elements into the host plant (Hall, 2002). The excess of Cr, Cu, and Ni was sequestered into the vesicles of arbuscular mycorrhizas (AM) living in the roots of C. dactylon grown in gold (Au) and uranium (U) mine tailings, which decreased trace element availability and toxicity to the host (Weiersbye et al., 1999). Inoculation of B. decumbens with a mix of AM fungi (Acaulospora morrowiae, Gigaspora albida, and Glomus clarum) inhibited Cd, Cu, and Zn translocation from the roots to the shoots by retaining these elements at the level of the roots and maintaining the trace element concentrations at below toxicity-critical concentration (Soares and Siqueira, 2008). Similarly, the inoculation of C. dactylon with two AM fungi (Funneliformis mosseae and Septoglomus constrictum) decreased both the Pb TF and the Pb bioconcentration factor (BCF) (Mahohi and Raiesi, 2021). Nonetheless, the beneficial effects of AM fungi on C. dactylon growth can be affected by the strong antagonistic interaction between AM fungi and plant growth-promoting rhizobacteria in polluted soils in the vicinity of Pb-mining sites (Mahohi and Raiesi, 2021).
The mechanisms of mycorrhizal associations that confer increased trace element tolerance to their host plants have proven to be difficult to resolve and may be diverse and present considerable plant species and trace element specificity since large differences in response to trace elements have been found between fungal species and to different trace elements within species (Hall, 2002). For instance, AM fungi colonization strongly correlated with the species A. capillaris, Arrhenatherum elatius, and Calamagrostis epigeios, and poorly correlated with the chemical characteristics of soil polluted with As and Pb (Teodoro et al., 2020). Although mycorrhizal associations can enhance plant tolerance to trace elements, studies about it in grasses are scarce, being an important gap to be closed in the future.
The Effect of Root Exudates on Trace Element Availability and Uptake
Plants secrete numerous metabolites from their roots into the rhizosphere to change the pH or to form trace element-metabolite complexes to manage nutrient bioavailability and cope with environmental trace element stresses (Chen et al., 2017). These exudates are a complex mixture of inorganic ions, gaseous molecules [e.g., carbon dioxide (CO2), hydrogen gas (H2)], and mainly carbon-based compounds that can be subdivided into low-molecular-weight compounds including amino acids, organic acids, sugars, and phenolics, and high-molecular-weight compounds that include mucilage and proteins (Kushwaha et al., 2016; Chen et al., 2017). Malinowski et al. (2004) aimed to identify the effects of endophytic bacterial strains and phosphorus (P) nutrition on Cu acquisition by F. arundinacea and observed that Cu2+-binding activity by the root exudates of this grass was not affected by endophyte infection but was higher (i.e., lower concentration of free Cu2+) in the absence of P.
The accumulation of Cd, Cu, and Pb by Echinochloa crus-galli grown in soils polluted with 600 mg Pb kg–1 soil, 40 mg Cd kg–1 soil, and 100 mg Cu kg–1 soil was 2- to 4-fold higher when this grass was assisted with root exudates from Belamcanda chinensis for 4 weeks (Kim et al., 2010). The root exudates also increased the BCF and TF for Cd, Cu, and Pb, which indicates that root exudates can have an important role in phytoextraction efficiency. However, the Cd bioavailability decreased due to malate exudation by S. bicolor exposed to Cd (0, 0.5, and 5 mg L–1 in solution) (Pinto et al., 2008), supporting the idea that the trace element-binding capabilities of root exudates can be important for trace element phytostabilization. The secretion types and patterns of root exudates vary in the function of plant species, physiological conditions, and soil environment (Chen et al., 2017), and apparently, in the function of these conditions, root exudates can contribute to phytoextraction (mainly of sites with a low level of trace elements pollution) or phytostabilization (Pinto et al., 2008; Kim et al., 2010). Indeed, trace element uptake can be restricted by immobilizing trace elements, limiting their passage through the plasma membrane (Hall, 2002), or favored by the formation of chelates, facilitating uptake in the cell and translocation of trace elements from the roots to shoots (Kushwaha et al., 2016). Nonetheless, studies about the role of root exudates in trace element tolerance in grasses considered for phytoextraction or phytostabilization are largely lacking. Most of the existing studies have focussed on the role of root exudates in Fe and Zn uptake by grasses in the context of plant mineral nutrition (Chen et al., 2017). Cakmak et al. (1996), for instance, reported that the release of phytosiderophores by Zn deficient A. orientale increased Zn uptake.
The Functions of Cell Walls in Tolerance Mechanisms
Once trace elements have crossed the barrier provided by mycorrhiza or have not been chelated by root exudates, they face another possible ‘line of defense’ against trace element-induced toxicity. Cell walls tend to bind divalent and trivalent trace element cations during their uptake by cells and at the final stage of their sequestration from the cytosol to decrease the toxicity due to free trace element into the cells (Hall, 2002; Krzesłowska, 2011). Almost 60% of Cd retained in the roots of P. maximum and B. decumbens was situated in the root apoplast, probably adsorbed to the cell walls (Rabêlo et al., 2021). Similarly, in P. australis, most Zn accumulated within the root apoplast and Zn concentrations followed the gradient: intercellular spaces > cell walls > vacuoles > cytosol (Jiang and Wang, 2008). Most of the Pb absorbed by B. decumbens was present in the root apoplast as chloropyromorphite, which thickened the cell walls surrounding these crystalline Pb deposits, indicating that the sequestration of insoluble Pb into the cell walls represents an important mechanism of Pb tolerance (Kopittke et al., 2008). Divalent (e.g., Cd2+, Pb2+, and Zn2+) and trivalent trace element cations can bind to functional groups of the cell wall, such as carboxyl (–COOH), hydroxyl (–OH), and –SH (Kushwaha et al., 2016).
Both, the primary and secondary cell walls have a range of mechanisms that can be customized to cope with trace elements. Pectins contain most of the negative charges within the primary cell wall and can sequester trace elements efficiently (Krzesłowska, 2011; Loix et al., 2017). Binding studies with low-methylesterified pectins showed that the affinity of divalent and trivalent trace element cations depends on the origin of pectins. Among the bivalent trace element cations, Cu2+ and Pb2+ are more strongly bound to pectins, whilst the binding of Zn2+ and Ni2+ is less strong (Krzesłowska, 2011). However, the type II of the primary wall and secondary wall, characteristic mainly for grasses, normally contain much fewer pectins (Pelloux et al., 2007). Pectins had no significant contribution to Cu binding in the cell wall of Paspalum notatum (Nabais et al., 2011). However, Ni was substantially bound to pectins in young roots (21 days), but not in older roots (120 days) (Nabais et al., 2011), probably due to a higher proportion of secondary cell walls in the older roots. The incrustation of phenolic components such as lignin during the formation of the secondary cell wall is an important process for the immobilization of trace elements and to create a stronger barrier for their entry (Krzesłowska, 2011; Loix et al., 2017). The amount of the cell wall-bound phenolics in V. zizanioides increased due to Cr, Cu, Ni, Pb, or Zn exposure compared with the non-exposed control conditions. This was different from what occurred after As exposure which caused the most severe phytotoxicity in this species (Melato et al., 2012). The genes involved in the lignin synthesis were upregulated due to Cd exposure in both the roots and shoots of P. americanum × purpureum (Zhao J. et al., 2019). An increased lignification and thickening of the cell layers of endodermis and exodermis in the root tissues and the cell walls of the xylem and cortical parenchyma was reported in B. decumbens exposed to Cd, Cu, Pb, and Zn (Gomes et al., 2011) and P. maximum exposed to Cd (Rabêlo et al., 2018c). Changes in the cell wall composition contribute to the trace element tolerance in both excluder and accumulator species, but the affinity of the trace element to the root cell wall tends to be different between them. Trace elements bound to the root cell wall of accumulators remain more available for xylem loading than in excluders or non-accumulator plants (Li et al., 2007).
The Role of Plasma Membranes and Trace Element Efflux
The role of the plasma membrane in trace element tolerance can either be by reducing the uptake of trace elements or by the increased efflux pumping of the trace elements that entered the cytosol (Hall, 2002). In this section, only the role of the plasma membrane in trace element efflux is addressed. After being taken up in the cells, a trace element can either be returned to the soil solution or remain in the apoplast. The direction of trace element efflux corresponds to the trace element accumulation phenotype of the plant. For instance, in non-accumulator plants, root efflux transporters direct trace elements to the soil solution, whilst in accumulators, the efflux system is directed toward loading trace elements into the xylem, on their way to the shoots (Lin and Aarts, 2012). With the objective to decrease As toxicity, P. clandestinum exposed to AsO43– (1, 5, 10, and 20 μmol L–1) reduced the AsO43– into AsO33– and realized a fast AsO33– efflux from the roots to the nutrient solution (Panuccio et al., 2012). Likely, P. clandestinum is a non-accumulator species for As. Although a fast AsO33– efflux was observed, no transporters involved in As efflux were reported. Some transporters can act in trace element efflux, such as transporters of the P1B-ATPase and ATP-binding cassette (ABC) families (Lin and Aarts, 2012), but information about their role in grasses is not available. The expression of ABC genes in the roots and shoots of F. arundinacea exposed to Ni was reported to be higher compared with the control (Shabani et al., 2016), but no more details were provided.
Chelation of Trace Elements by Ligands and Transport Into Vacuoles
Organic acids, amino acids, and (poly)peptides are potential ligands for trace elements and thus, can play a role in trace element tolerance and detoxification (Cobbett and Goldsbrough, 2002; Sharma and Dietz, 2006; Osmolovskaya et al., 2018). The role of organic acids in the intracellular detoxification of trace elements has been studied most intensively in hyperaccumulators, especially in Zn hyperaccumulator species (Osmolovskaya et al., 2018). In such plants, Zn is chelated by malate to form a relatively weak complex in the cytosol that is transported into the vacuoles, where the complex dissociates and Zn2+ binds with stronger chelators such as citrate or oxalate. Malate is re-exported to the cytosol (Osmolovskaya et al., 2018). The involvement of malate, citrate, and oxalate in trace element tolerance was also demonstrated in non-hyperaccumulators such as L. perenne, which presented increased concentrations of these organic acids in the shoot under Ni exposure (Yang et al., 1997). Oxalate may participate in Cd sequestration into the vacuole in roots and also in Cd translocation to the shoot in S. alternifloria (Chai et al., 2012). On the other hand, the concentrations of organic acids such as malate, citrate, and fumarate were not higher in the roots, stems, or leaves of Cd-exposed P. maximum than in non-exposed ones (Rabêlo et al., 2018b). Probably other mechanisms of tolerance to Cd such as enhanced synthesis of amino acids, GSH, and PCs are more important than organic acids for Cd detoxification in P. maximum (Rabêlo et al., 2019).
Upon exposure to toxic concentrations of trace elements, plants often synthesize a range of diverse metabolites that accumulate to concentrations in the millimolar range, particularly specific amino acids, such as proline and histidine (Sharma and Dietz, 2006). An increased accumulation of proline was described in the leaves of P. maximum exposed to Cu (Gilabel et al., 2014) and C. dactylon exposed to Cd (Xie et al., 2014). The proline-inducing ability of different trace elements varies, and when compared at equimolar concentrations in the growth medium, Cu is the most effective in inducing proline accumulation followed by Cd and Zn, respectively (Schat et al., 1997). Proline functions as an osmolyte, radical scavenger, electron sink, stabilizer of macromolecules, and a cell wall component (Sharma and Dietz, 2006). Trace element-tolerant plants, like Zn-tolerant ecotypes of Deschampsia cespitosa, possess substantially elevated constitutive proline levels in different plant parts (Smirnoff and Stewart, 1987). Some studies also reported increased histidine concentrations induced by toxic concentrations of trace elements. Nickel exposure led to higher histidine concentrations in the roots of D. cespitosa (Nguyen et al., 2018), while histidine concentrations increased in the roots, stems, and leaves of P. maximum exposed to Cd (Rabêlo et al., 2018b). Histidine can also bind other divalent ions such as Cu and Zn, decreasing their toxicity and the level of induction of oxidative stress in the cytosol (Zheng et al., 2013). However, the chelation of trace elements (e.g., Ni) by free histidine accumulated in the roots inhibits the trace element sequestration into the vacuole and promotes trace element translocation from the roots to shoots (Richau et al., 2009). Besides proline and histidine, other amino acids such as cysteine can be more accumulated under trace element exposure in grasses (Rabêlo et al., 2017b), probably due to their use for peptides synthesis.
Phytochelatins and MTs are the best characterized and probably the most important trace element-binding ligands in plant cells (Cobbett and Goldsbrough, 2002). Phytochelatins act on trace element chelation and are involved in their transport from the cytosol to the vacuole and also in its translocation from the roots to shoots (Cobbett and Goldsbrough, 2002; Mendoza-Cózatl et al., 2008). In the roots and stems of P. maximum, the synthesis of PCs was strongly increased by Cd exposure (Rabêlo et al., 2018b). Phytochelatins are synthesized inductively by exposure to not only Cd but also by other trace elements such as Costal, Cu, Hg, Pb, and Zn (Yadav, 2010), as reported in the roots of L. perenne exposed to As (Schulz et al., 2008) and V. zizanioides exposed to Pb (Andra et al., 2010). The processes involved in the transport of trace element-PCs complexes from the cytosol to the vacuole were reviewed in detail (Sharma et al., 2016). Although PCs are important players in trace element detoxification, they are not always induced under trace element exposure due to the combined action of other detoxification processes (Rabêlo et al., 2019), as observed in D. cespitosa exposed to Ni (Hayward et al., 2013) and B. decumbens and P. maximum exposed to Cd (Rabêlo et al., 2021). Besides the PCs, MTs are supposed to function as trace element-binding ligands in plant cells (Cobbett and Goldsbrough, 2002), although their role in plants remains to be confirmed. They could play a role in trace element metabolism, but their precise function is not clear; they may have distinct functions for different trace elements; alternatively, they could function as antioxidants, although evidence is lacking, while a role in plasma membrane repair is another option (Hall, 2002). The expression of MET genes that encode for MTs increased in the roots and shoots of F. arundinacea exposed to 30 mg Ni kg–1 soil compared with non-exposed plants (Shabani et al., 2016). Ukoh et al. (2019) observed that the MTs concentration increased in the roots and stems of P. maximum exposed to 5 mg Zn kg–1 soil compared with the non-exposed controls, but there was no MTs increase in the leaves.
Trace elements not bound to chelators also can be transported to vacuoles through a series of transporters (Sharma et al., 2016). However, studies about this are scarce in grasses. To the best of our knowledge, only two studies showing the role of the vacuolar Na+/H+ antiporter (NHX1) and the expression of HMA3, a P1B-ATPase, in Cd transport from cytosol to vacuoles in Leptochloa fusca and Festulolium loliaceum, respectively, are currently available (Adabnejad et al., 2015; Guo et al., 2017).
Antioxidative Machinery in Relation to Trace Element Toxicity
Oxidative stress is considered as a primary effect of the exposure to toxic concentrations of trace elements (e.g., Cd) (Clemens, 2006; Cuypers et al., 2016). This scenario occurs when there is a serious imbalance in any cell compartment between the production of ROS (e.g., O2⋅–, H2O2, and ⋅OH) and antioxidative defense. This can be initiated due to the insufficient efficiency of the tolerance mechanisms described above (Gratão et al., 2005). According to Hall (2002), trace element-tolerant plants do not appear to possess enhanced tolerance to free radicals or ROS, but rather, rely on improved mechanisms for trace element homeostasis. For instance, enzymes involved in ROS scavenging [e.g., superoxide dismutase (SOD) (EC 1.15.1.1), catalase (CAT) (EC 1.11.1.6), and ascorbate peroxidase (APX) (EC 1.11.1.11)] are not principal in the oxidative stress control in the roots, stems, and leaves of P. maximum that is considered as a Cd-tolerant non-hyperaccumulator species, since it can promote the vacuolar sequestration of Cd from the cytosol (Rabêlo et al., 2018b,d). This suggests that plants that are not equipped with a trace elements tolerance mechanism need a higher antioxidative capacity (non-enzymatic and enzymatic) to cope with trace element-induced stress than trace element-tolerant plants. Several non-enzymatic antioxidants such as ascorbate, GSH, flavonoids, alkaloids, tocopherols, carotenoids, phenolic compounds, and non-protein amino acids are employed by plants for ROS scavenging (Gratão et al., 2005). Among them, GSH, which can be oxidized to oxidized glutathione (GSSG) avoiding oxidative damages in sensitive organelles, is considered the principal non-enzymatic antioxidant in plants (Yadav, 2010; Seth et al., 2012). Moreover, GSH is used as a substrate for PCs synthesis and can also act as a ligand for trace elements, besides transmitting specific information tuning cellular signaling pathways under trace element stress conditions (for a comprehensive review see Seth et al., 2012). The beneficial effects of GSH on trace element tolerance were described in L. perenne grown in soil polluted with As, Cu, Hg, Pb, and Zn (Anjum et al., 2013), Poa pratensis exposed to Cu (Liu et al., 2016), and both P. maximum and B. decumbens exposed to Cd (Rabêlo et al., 2021). Under high Cd accumulation, symptoms of oxidative stress, such as lipid peroxidation, can occur as a consequence of GSH depletion due to the binding of Cd2+ to GSH and the formation of GSH-derived PCs (Clemens, 2006). Sugars such as galactinol and raffinose that exhibit a similar capacity as GSH to scavenge ⋅OH radicals (Nishizawa et al., 2008) were also shown to be important in oxidative stress control in grasses like P. maximum exposed to Cd (Rabêlo et al., 2018b,d). The major ROS-scavenging mechanisms of plants include enzymes of the AsA-GSH cycle as SOD that dismutates O2⋅– to H2O2, and CAT, APX, and guaiacol peroxidase (GPX) (EC 1.11.1.7) that are involved in reducing H2O2 into H2O (Gratão et al., 2005). Ullah et al. (2020a) reported that, after 90 days of growth, the SOD activity was enhanced in the leaves of B. mutica and L. fusca exposed to Cd (10 and 50 mg Cd kg–1 soil) or Pb (50 and 250 mg Pb kg–1 soil) compared with the non-exposed plants. Similarly, the SOD activity increased in both the roots and shoot of V. zizanioides exposed to AsO43– or AsO33– (0, 25, 50, 100, 200, 400, and 800 μmol L–1) for 28 days (Yu et al., 2020). In contrast, the activities of Mn-SOD and Cu/Zn-SOD did not increase in the roots, stems, and leaves of P. maximum exposed to Cd (100 μmol L–1) for 9 days (Rabêlo et al., 2018d).
The activity of the enzymes of the AsA-GSH cycle is quite variable in grasses and depends on the concentration of the trace elements, exposure time, environmental conditions, plant nutritional status, and the action of other tolerance mechanisms (Rabêlo and Borgo, 2016; Rabêlo et al., 2018a). The activity of APX in the leaves of P. pratensis increased due to Cu exposure (Liu et al., 2016), as well as the glutathione reductase activity (GR) (EC 1.6.4.2), which reduces GSSG into GSH; monodehydroascorbate reductase (MDHAR) (EC 1.6.5.4) and dehydroascorbate reductase (DHAR) (EC 1.8.5.1) that act on AsA recycling (Gratão et al., 2005). However, the activities of CAT, GPX, and GR did not enhance in the L. perenne simultaneously exposed to As, Cu, Hg, Pb, and Zn (Anjum et al., 2013). These findings indicate that specific aspects related to plant species and tissues must be considered to interpret antioxidative responses under trace element exposure. A simplified and general summary of the potential cellular mechanisms involved in trace element detoxification and tolerance is presented in Figure 4 for both excluder and accumulator grasses used for trace elements phytoremediation.
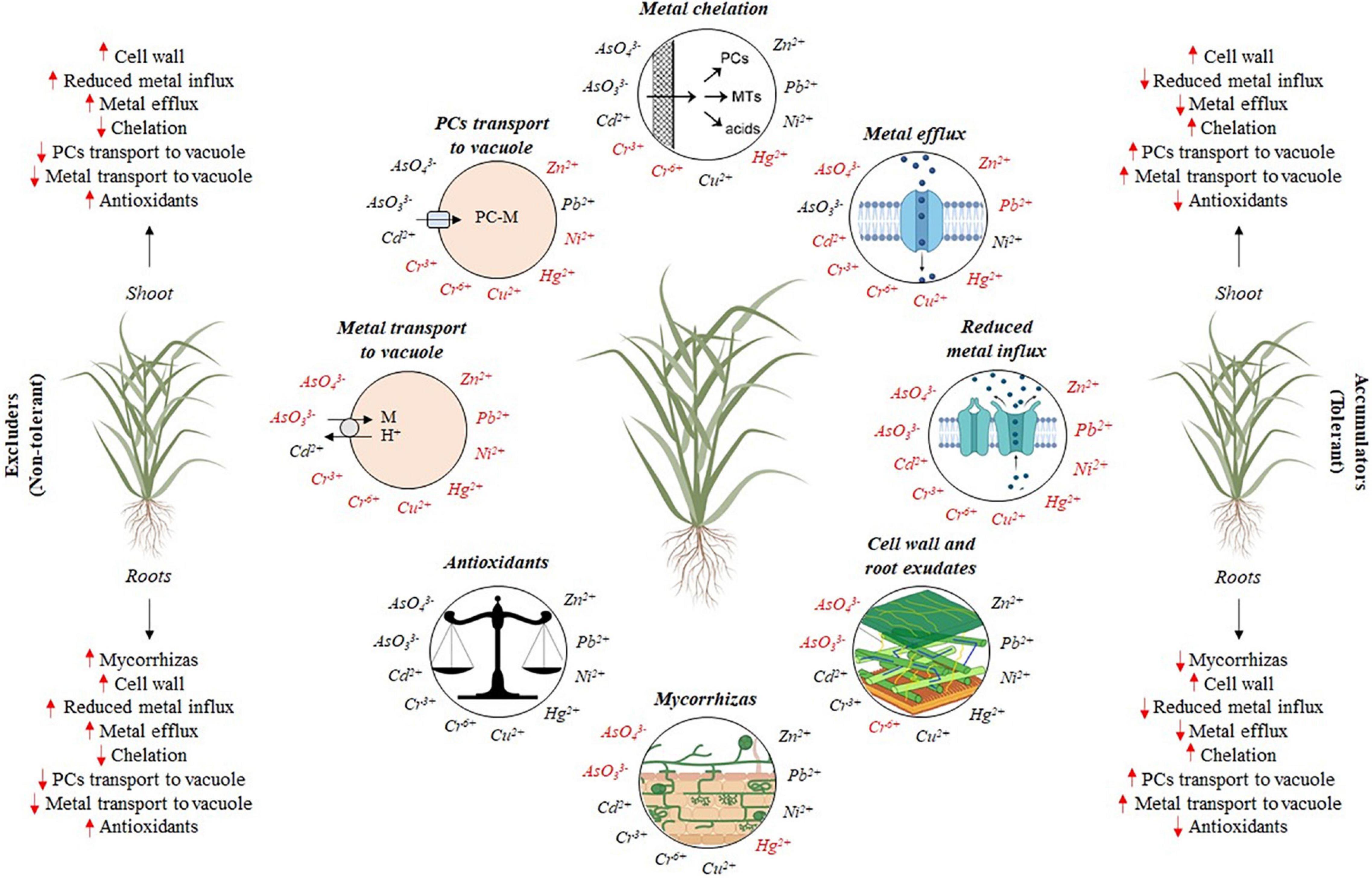
Figure 4. Summary of cellular mechanisms involved in metal detoxification and tolerance in grasses: (i) restriction of metal movement to roots by mycorrhizas; (ii) binding to the cell wall and root exudates; (iii) reduced influx across plasma membrane; (iv) active efflux into the apoplast; (v) chelation in the cytosol by phytochelatins (PCs), metallothioneins (MTs), amino acids and organic acids; (vi) transport of PC-M complex into the vacuoles; (vii) transport and accumulation of metal (M) not chelated into the vacuole; (viii) the balance between antioxidants and oxidants. Adapted from Hall (2002). Red arrows indicate which tolerance mechanisms are possibly more (↑) or less (↓) requested by excluder and accumulator grasses. Metals written in red indicate the need for further studies in relation to each specific tolerance mechanism or indicate that the tolerance mechanism is not employed in its detoxification.
Use of Grasses for Trace Element Phytoremediation
Plants can be divided into four groups with respect to their responses for uptake and tolerance to trace elements: (i) “regular” plants only tolerate low concentrations of available trace elements in the soil before they die due to phytotoxicity; (ii) excluders can grow over a wide range of available phytotoxic trace elements before physiological mechanisms lose control and allow for unregulated uptake, resulting in the death of the plant; (iii) bioindicators take up trace elements over a wider range than “regular” plants and the concentrations in the plant leaves reflect those of the soil until phytotoxicity prevents further growth and causes the death of the plant; and (iv) (hyper)accumulators can tolerate much higher concentrations of bioavailable trace elements than “regular” plants, bioindicators, and excluders (Baker, 1981; van der Ent et al., 2013). The most interesting groups for phytoremediation purposes are excluders, which normally accumulate trace elements in the roots withy very limited translocation to the shoots (Awa and Hadibarata, 2020) and are recommended for phytostabilization (Alkorta et al., 2010), and (hyper)accumulators, which preferentially accumulate trace elements in the shoot (Awa and Hadibarata, 2020) and can be used for phytoextraction (Vangronsveld et al., 2009). Bioindicators with a high biomass yield can be used also for phytoextraction in moderately polluted agricultural soils (Rabêlo et al., 2020a). Most grass species are trace element excluders (Fernández et al., 2017), but there exist many bioindicators and a few accumulators as well. Both excluders and accumulators tolerant to hypoxia can also be used to remove trace elements from polluted wastewaters, groundwaters, and surface waters; this approach is known as rhizofiltration or phytofiltration (Awa and Hadibarata, 2020). In order to screen grasses according to their use for trace element phytoremediation, we briefly described below the main aspects associated with phytoextraction, phytostabilization, and phytofiltration, and examples of appropriate species for each strategy, considering the fact that there exists trace element specificity within most grass species. Therefore, the same species could be a suitable candidate for the phytoextraction of trace element x and phytostabilization of trace element y, or in some cases (e.g., A. capillaris) could be a suitable candidate for both phytoextraction and phytostabilization of the same trace element depending on the composition of its rhizosphere microbiome (Truyens et al., 2014).
Phytoextraction
It is necessary to consider that there exist important limitations for trace element phytoextraction: (i) it can only be applied to low to moderately polluted soils, and (ii) its applicability depends on the depth of rooting, which varies with the plant species used, the soil type, and the depth of the groundwater table, but on average is not more than 50 cm (Vangronsveld et al., 2009). An ideal plant for trace element phytoextraction should possess the following characteristics: (i) tolerant to the trace element accumulated, (ii) fast-growing and high biomass, (iii) accumulating trace elements in the above-ground parts, and (iv) easy to harvest (Vangronsveld et al., 2009). Furthermore, another point requiring caution when choosing plant species for phytoextraction purposes is that a non-specific “breakthrough” of trace element uptake into the shoots may occur when the tolerance limit of an excluder species is exceeded (Baker, 1981). However, this does not imply that such species can be considered as an (hyper)accumulator if the high concentration of the trace element absorbed results in the death of the plant (van der Ent et al., 2013). Most (hyper)accumulators do not occur on non-metal-enriched soils due to the competitive disadvantages and greater sensitivity to fungal and pathogen infections (van der Ent et al., 2013) unlike most of the grasses listed as suitable choices for trace element phytoextraction in Table 2. Given that most, grass species also occur on “normal” soils, they are pseudometallophytes (Tordoff et al., 2000). Probably, most grass species that have been assessed for trace element phytoextraction are actually bioindicator plants, for example B. decumbens and P. maximum (Rabêlo et al., 2020a). Thus, the use of such plants for trace element phytoextraction is feasible only in mildly polluted agricultural soils, otherwise trace element-induced phytotoxicity will prevent further growth and biomass yield. In the latter case, the use of grasses used for trace element phytoextraction will be very inefficient, especially if compared to hyperaccumulators.
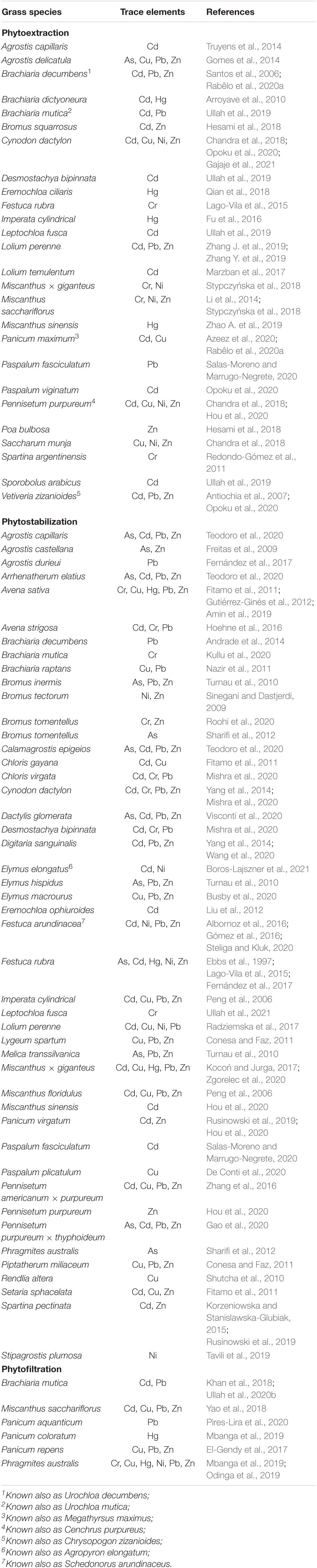
Table 2. Examples of suitable grass species for each strategy of phytoremediation in sites contaminated with potentially toxic trace elements.
Theoretical calculations indicate that only grasses producing more than 75 t DW ha–1 yr–1 are as efficient as Noccaea caerulescens (a known Cd hyperaccumulator) to reduce Cd concentrations in mildly polluted soils (Figure 5A). Furthermore, the use of grasses producing < 15 t DW ha–1 yr–1 results in a significant reduction in the concentrations of Cd, Ni, and Zn in polluted soil only after 10 cultivation cycles which differs greatly compared to hyperaccumulators (Figures 5A–C). However, it is necessary to be cautious in interpreting the results of such theoretical calculations because the values used for biomass yield (t DW ha–1 yr–1) and trace element concentrations were for grasses listed as good candidates for trace elements phytostabilization (Table 2) and suitable data were not found in the same study for phytoextractor grasses. In this respect, the efficiency of grasses listed as good candidates for phytoextraction to reduce trace element concentrations might be closer to a hyperaccumulator, but again, only for mildly polluted soils. In this scenario, some authors have mentioned that effective trace element removal realized by harvesting non-hyperaccumulator plants with high biomass yield is not different from the removal by hyperaccumulators that generally produce less biomass (Ebbs et al., 1997; Kayser et al., 2000). On the other hand, in soils that are more heavily polluted, trace element removal by hyperaccumulator plants is always higher compared with plants with higher biomass (Chaney et al., 1997) due to the phytotoxicity-induced decline of the biomass production of the latter.
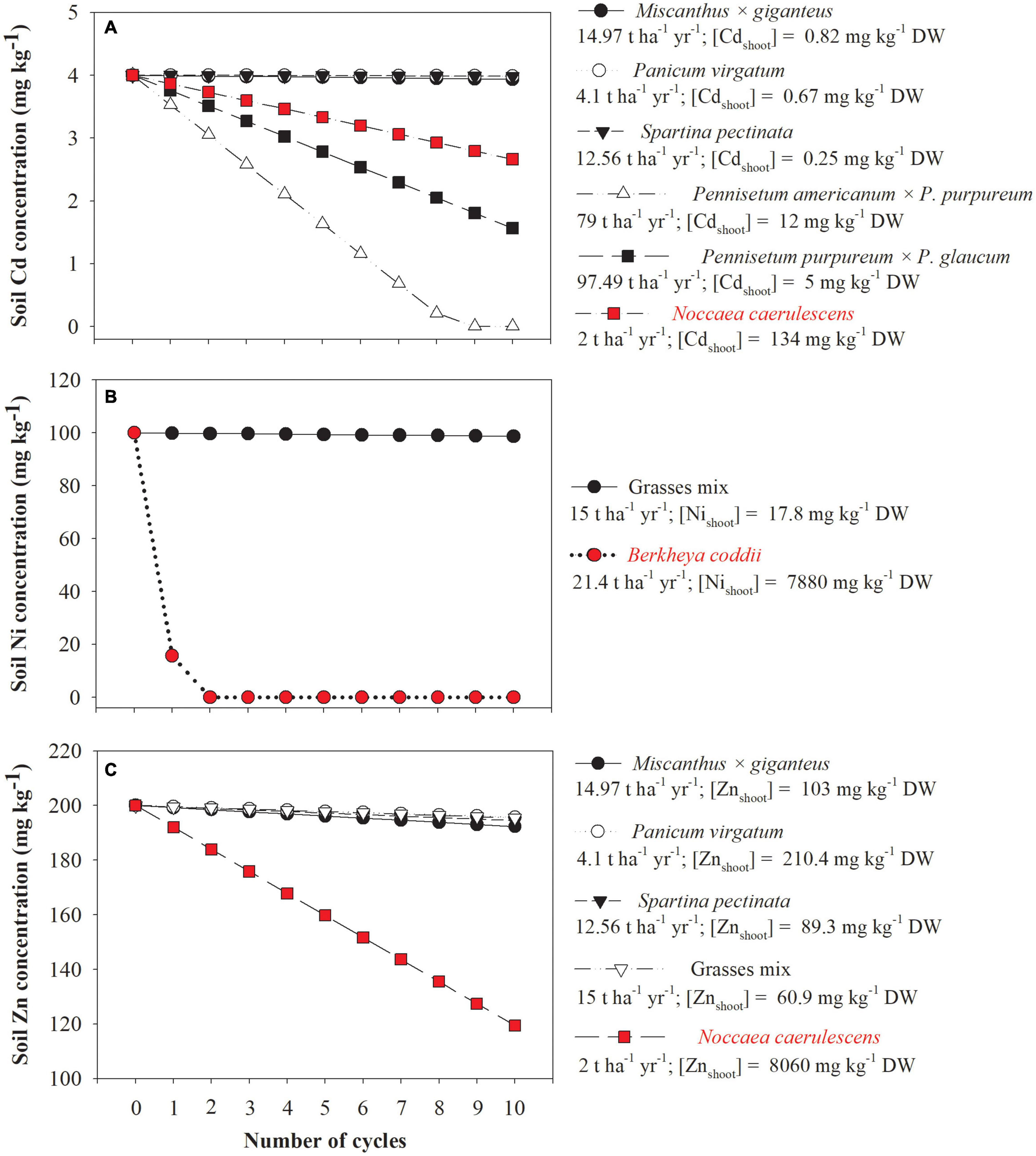
Figure 5. Theoretical reduction on cadmium (Cd, A), nickel (Ni, B), and zinc (Zn, C) concentrations by the cultivation of grasses and known hyperaccumulator plants (highlighted in red color), in a mildly contaminated soil, according to the number of cycles of cultivation (in this specific case, one cycle corresponds to 1 year of cultivation). The assumptions made were: (i) the total soil metal concentration decreases linearly due to a constant yearly extraction, (ii) the contamination and rooting depth are 0.2 m, and (iii) soil density is 1 kg dm–3. Grasses mix = Festuca arundinacea, Festuca rubra, Lolium perenne, and Poa pratensis. The data of the biomass yield and metals concentration used to plot this figure were extracted from Robinson et al. (1997); Zhang et al. (2014), Kitczak et al. (2016); Jacobs et al. (2018), Rusinowski et al. (2019), and Zhou et al. (2020).
Phytoextraction efficiency is often estimated by calculating the BCFs and TFs. It has been suggested that only plant species with both factors > 1 have potential to be used in phytoextraction (Buscaroli, 2017). In this case, the available concentration of the trace elements must be used for calculating the BCF, since the use of (pseudo)total soil concentrations can result in a very distorted representation of the actual viablity especially for trace elements with generally low bioavailability, such as Cr and Pb (Senila, 2014; Ertani et al., 2017). Another point requiring caution to interpret BCFs is that high (total or bioavailable) trace element concentrations in the soil may result in a BCF < 1, but even so, the plant species might be very efficient in sequestering the trace element, but the opposite can also occur (van der Ent et al., 2013). Given this scenario, the use of TF can provide a higher reliability to screen plant species (grasses or not) for their potential phytoextraction capacities since this simple calculation allows for the discrimination between excluder and accumulator plants. Moreover, another point that should be considered when screening the suitability of grass species for phytoextraction is their biomass yield. Besides high BCF and TF, high phytoextraction efficiencies are also directly correlated with high biomass (McGrath and Zhao, 2003), and therefore this parameter should be considered, as shown in Figure 5A.
Phytostabilization
Phytostabilization is not a technology for the clean-up of polluted soils, but rather a management strategy for stabilizing (or deactivating) elements that are potentially toxic (Vangronsveld et al., 2009). Revegetation stabilizes the polluted soil, establishing a cover that prevents the dispersion of pollutants by water or wind erosion and reduces the mobility and bioavailability of trace elements (Alkorta et al., 2010), thus preventing or restricting their entry into the food chains (Buscaroli, 2017). Plants can immobilize trace elements in soils through sorption by roots, precipitation, complexation, or changing the trace element valence in the rhizosphere (Alkorta et al., 2010). The use of soil amendments (e.g., liming, clays, and zeolites) to support plant growth, including grasses (Gao et al., 2020) can increase phytostabilization efficiency (Vangronsveld et al., 2009).
The choice of plant species is a crucial aspect of trace element phytostabilization. Ideally, plants should develop an extensive root system and large biomass in the presence of high concentrations of trace elements whilst keeping the root-to-shoot translocation as low as possible (Alkorta et al., 2010). In this respect, root colonization by AM fungi can have a great effect on the phytostabilization capacity of grasses such as A. capillaris, A. elatius, and C. epigejos exposed to As, Cd, Pb, or Zn by restricting the entry of trace elements into the host plant (Teodoro et al., 2020). Similarly, the application of soil amendments such as biochar, peat, manure, and even non-polluted soil, has been shown to efficiently keep the BCFs of As, Cd, Pb, and Zn < 1 in the grass P. purpureum × thyphoideum by decreasing their bioavailability (Gao et al., 2020). Based upon these empirical results, it is clear that assisted phytostabilization is indeed promising, but even so, the plant species selected for trace elements phytostabilization should ideally present both a BCF and a TF < 1 (Buscaroli, 2017). Further details about phytostabilization prospects have been presented by Alkorta et al. (2010). An overview of the most suitable grasses to perform trace element phytostabilization is presented in Table 2.
Phytofiltration
Phytofiltration employs plant roots to absorb pollutants in the root zone, concentrating and precipitating them onto or within their harvestable root mass (Kristanti et al., 2021). The site of trace elements sorption (on roots, within roots, or in the aerial organs of plants) depends on the type of plant and the speciation and concentration of the specific trace element concerned (Kristanti et al., 2021). Suitable plants selected for trace element phytofiltration should ideally be tolerant to hypoxia and the target trace element(s) and possess an extensive root surface area for sorption (Awa and Hadibarata, 2020). Grasses well adapted to conditions of low dioxygen (O2) availability such as B. mutica and P. australis can be good candidates for trace elements phytofiltration. Brachiaria mutica persisted in a hydroponic system containing high Pb concentrations (100, 200, and 500 μmol L–1) and retained the major part of Pb ad- and absorbed in its roots (Khan et al., 2018). Phragmites australis efficiently decreased the concentrations of Cr, Cu, Ni, Pb, and Zn in a phytofiltration system for wastewaters (Odinga et al., 2019). Terrestrial plants normally have extensive fibrous roots that provide a larger surface area for trace elements sorption than aquatic plants (Awa and Hadibarata, 2020; Kristanti et al., 2021). However, V. zizanioides exposed to landfill leachate were shown to accumulate high concentrations of Cr, Ni, and Zn (Fasani et al., 2019). Nonetheless, its growth under leachate exposure was strongly dependent on leachate composition, making a case-by-case evaluation of plant tolerance necessary before any large-scale application. Moreover, the exposure time, ambient temperature, and pH of the water to be treated also have a primary influence on the responses of such plants used for any phytofiltration system (Kristanti et al., 2021). From a broad consideration of the issues mentioned in this section, it emerges that currently, only a few grass species presented satisfactory results for their application in trace element phytofiltration (see Table 2).
Strategies to Improve the Efficiency of Trace Element Phytoremediation by Grasses
Although several grass species are suitable to be used for phytoremediation purposes (Table 2), considering the particularities mentioned above, the use of non-hyperaccumulator species to lower trace element concentrations in the environment to acceptable levels often takes a long time, in the order of decades or even centuries (Vangronsveld et al., 2009). For example, 22 and 26 years would be needed to reduce the pseudo-total Cd concentration of 3.6 mg kg–1 soil to 0.5 mg kg–1 soil (background reference values for São Paulo State, Brazil), using P. maximum and B. decumbens, respectively (Rabêlo et al., 2020a). Toxicity induced by trace elements compromises not only the capacity of the plant to remove trace elements, but also its capacity to stabilize polluted soils (Chen and Wong, 2006; Gao et al., 2020). Therefore, there remains a general need for the improvement of extraction and stabilization efficiencies. Some strategies can enhance phytoextraction and phytostabilization efficiencies by changing the plant availability of trace elements and/or increasing the trace elements tolerance of the plants, such as (i) the optimization of agronomic practices (e.g., by supplying nutrients and beneficial); (ii) optimizing the plant-microorganism interactions; (iii) changing the plant growth conditions (e.g., using soil amendments); (iv) by genetic breeding to overcome the disadvantages of phytoremediation (Salt et al., 1998; Koźmińska et al., 2018; DalCorso et al., 2019).
Nutrients and Beneficial Elements Supply
In addition to improving plant growth, an appropriate supply of nutrients [nitrogen (N), P, potassium (K), Calcium (Ca), Mg, S, boron – B, chlorine (Cl), Cu, Fe, Mn, molybdenum (Mo), Ni, and Zn] and beneficial elements [cobalt (Co), sodium (Na), selenium (Se), and silicon (Si)], can also alleviate different growth stresses, including trace element-induced stress (Sarwar et al., 2010). Depending on the situation (e.g., plant species, growth conditions, and low level of pollution by trace elements), optimizing the nutritional status can contribute to improving phytoextraction or phytostabilization efficiencies. Many studies have been conducted to assess mainly the effects of N, S, and Si supplementation on the tolerance of grasses adopted for trace element phytoremediation.
Nitrogen can alleviate trace element toxicity by increasing chlorophyll contents and thus enhancing the photosynthetic capacity, supporting the higher synthesis of N-containing metabolites like amino acids and derivatives, GSH, and PCs, and by enhancing the activities of antioxidative enzymes (Sharma and Dietz, 2006; Sarwar et al., 2010; Singh et al., 2016), but for each trace element, these effects depend on specific combinations of ammonium (NH4+) and nitrate (NO3–). The supply of N as NO3– was reported to support Cu phytostabilization, whilst the combination of NH4+ (30%) and NO3– (70%) enhanced the activity of the antioxidative system of P. maximum and increased Cu phytoextraction (Souza Junior et al., 2019). The negative effects of Cd on photosynthesis and the balance between oxidants and antioxidants in P. maximum were also attenuated by the supply of combinations of NH4+ (50%) and NO3– (50%), which resulted in higher Cd phytoextraction (de Sousa Leite and Monteiro, 2019a,b).
Similarly, S can also alleviate trace element toxicity by promoting the synthesis of S-containing metabolites like GSH and PCs, enhancing the activity of the AsA-GSH cycle, and regulating ethylene signaling, among other factors (Sarwar et al., 2010; Singh et al., 2016). An appropriate S supply allowed higher Cd translocation from the roots to the stems in P. maximum (Rabêlo et al., 2018c), as well as higher GSH and PCs synthesis, and improved the activities of enzymes of the AsA-GSH cycle, which is essential to lower Cd phytotoxicity and tiller mortality rate in grasses, which contributed to a higher Cd phytoextraction efficiency (Rabêlo et al., 2017a,b, 2018b). Panicum maximum appropriately provided with S was more tolerant to Cu-exposure (Gilabel et al., 2014).
Silicon can alleviate trace element toxicity through several mechanisms that include the regulation of trace element uptake and root-to-shoot translocation, modulation of the cation binding capacity of the cell walls, increase of enzymatic (e.g., SOD, APX, and DHAR) and non-enzymatic (e.g., AsA and GSH) antioxidants, and the complexation or co-precipitation of trace element ions with Si in the cytoplasm, followed by sequestration of the trace elements in the vacuoles (Pilon-Smits et al., 2009). This is of particular importance in monocots that show high Si accumulation (10–15%) (Hodson et al., 2005). Supplementation of Si lowered Cu translocation from the roots to the shoots in P. maximum, alleviating the Cu toxicity in the leaves, which improved the biomass production and allowed the successful harvesting of the aboveground biomass (Vieira Filho and Monteiro, 2020). Silicon also appears to play an important role in Cu tolerance in S. densiflora, not by avoiding its uptake by roots, but via lowering the Cu translocation from the roots to the leaves, resulting in a general decrease of Cu-induced deleterious effects on the photosynthetic apparatus (Mateos-Naranjo et al., 2015). Evaluating the influence of CaO-activated Si-based slag amendment on growth and Cd, Cr, Cu, Pb, and Zn uptake of V. zizanioides, Mu et al. (2019) found that the beneficial effect of Si depends on the dose and trace element speciation.
Plant–Microorganism Interactions
The interaction between plant roots and microorganisms can support phytostabilization through the association with AM fungi, and phytoextraction through the association with plant growth-promoting bacteria (PGPBs) (DalCorso et al., 2019). This section focuses on the possible role of PGPB since the effects of AM fungi on trace element uptake and plant tolerance to trace elements were already described above. Plant growth-promoting bacteria, such as phosphate and potassium solubilizers, free-living N2-fixing bacteria, and rhizobia, can promote plant growth and increase the biomass yield by the production of phytohormones [such as indoleacetic acid (IAA)], lowering the stress originating from ethylene production [by lowering the levels of 1-aminocyclopropane-1-carboxylic acid (ACC) due to ACC-deaminase (EC 3.5.99.7) activity], or by improving the plant nutritional status through N2 fixation, phosphate-solubilization, or siderophore production (Sessitsch et al., 2013). Plant growth promotion plays a major role in the extraction and removal of trace elements since the enhancement of biomass production results in a higher overall trace element yield (amount of phytoextracted trace element) (McGrath and Zhao, 2003; Sessitsch et al., 2013). The influence of 14 strains of Cd/Zn-resistant rhizosphere bacteria (isolated from Poaceae and woody trees/shrubs) was evaluated on the growth and trace elements accumulation of F. pratensis (Becerra-Castro et al., 2012). Almost all strains promoted the growth of this grass. In the same way, the potential use of Cd-resistant bacteria in P. purpureum × americanum was evaluated, and plants inoculated with Micrococcus sp. showed higher shoot biomass and Cd accumulation compared with non-inoculated plants after six months of growth (Wiangkham and Prapagdee, 2018). In addition to plant growth promotion, bacteria have been reported to have beneficial effects on plant stress tolerance, likely due to the enzyme ACC deaminase, leading to lower levels of stress-induced ethylene in the plant (Sessitsch et al., 2013). The bacterium Pseudomonas asplenii AC was genetically transformed to express a bacterial gene encoding the enzyme ACC deaminase, and both native and transformed bacteria were tested in P. australis. The inoculation of seeds with the transformed P. asplenii AC significantly increased the seed germination and enabled the plants (shoot and roots) to reach a greater size than non-inoculated plants when exposed to Cu (Reed et al., 2005). The cloning and expression of an ACC deaminase gene in an Azoarcus strain provided a recombinant strain that protected inoculated rice plants against Cd stress and increased the Cd concentration in the shoots (Fernández-Llamosas et al., 2020). The use of bacteria to support plants employed in trace element phytoremediation has proved to be advantageous, and it can be much more explored since up to 99% of the soil microbial taxa are yet to be cultured and investigated (Pham and Kim, 2012).
Utilization of Soil Amendments in Phytoremediation
The mobility and bioavailability of trace elements in the soil can be changed by the addition of either organic or inorganic amendments (Vangronsveld et al., 1995, 1996; Wiszniewska et al., 2016). Organic amendments are considered especially effective for Cr stabilization, whereas in the case of other trace elements (As, Cu, Pb, Cd, and Zn) they may have both positive and negative effects (Kumpiene et al., 2008). The addition of humic acid did not change the Cu and Zn bioavailability in soil affected by mining activities, but its use reduced Cu and Zn translocation in V. zizanioides, so that the combined use of this species with humic acid at 10–20 g kg–1 can be an effective strategy for both Cu and Zn phytostabilization in some mine soils (Vargas et al., 2016). The effects of two organic amendments (organic fertilizer – pig slurry and plant ash and sewage sludge) on the stabilization of Cd, Cu, Pb, and Zn in acidic soil established with P. americanum × purpureum, Euchlaena mexicana, or S. dochna were evaluated, and the use of 5% organic fertilizer only or combined with 5% sewage sludge decreased the trace element bioavailability and the trace element concentrations in the shoots (Zhang et al., 2016).
Some organic amendments, such as biochar and ethylenediaminetetraacetic acid (EDTA) were reported to increase phytoextraction instead of promoting trace elements phytostabilization (references). Although this result is desirable, caution is needed for its use in assisted grass growth since such plants are not efficient in performing phytoextraction in soils heavily polluted by trace elements. Therefore, the effects of soil amendments should be evaluated case-by-case (Wiszniewska et al., 2016). Combined use of biochar and PGPB was found to increase Cd uptake by 412% and the Cd BCF by 403% in V. zizanioides compared with the non-amended control (Wu et al., 2019). The EDTA application increased the Cr concentration in the roots and shoots, the Cr BCF, and Cr TF in P. americanus × purpureum, leading to the improper functioning of PSII, and a lower biomass yield due to Cr toxicity (Ram et al., 2019). These findings show that although organic amendments are generally considered as effective for Cr stabilization (Kumpiene et al., 2008), the opposite effect can occur due to differences in the growth medium.
The use of inorganic soil amendments such as lime, clay minerals, phosphorus-bearing materials, Mn oxides, and alkaline materials often decreases the trace element bioavailability, enhancing the trace element phytostabilization (Kumpiene et al., 2008; Wiszniewska et al., 2016). Liming was reported to be necessary to ensure the persistence of the grass C. dactylon grown in Cu-polluted soils, by lowering the Cu accumulation in its leaves due to a decreased plant availability of Cu produced by the increase of the soil pH (Shutcha et al., 2010). Similarly, the trace element-immobilizing agents, namely, cement, slag, phosphate rock, bitumen, Fe- and aluminum (Al)-gels, and synthetic Mn oxide (δ-MnO2) reduced the bioavailability of Cd and Pb in a soil covered with E. stagninum (Aboulroos et al., 2006). From the studies concerning organic and inorganic soil amendments, it is clear that the use of soil amendments for assisting the growth of grasses can improve both the phytoextraction and phytostabilization of trace elements, but the results largely depend on the soil conditions, the level of pollution, trace element speciation, plant species, etc. Therefore, the effects of soil amendments must be evaluated case-by-case, especially considering the fact that high trace element bioavailability induced by soil organic amendments such as biochar and EDTA can decrease plant growth and biomass yield, and lower phytoextraction efficiency. Moreover, limited information is available concerning the long-term effects of soil amendments on the soil microbiota and the metabolism of plants used for trace element phytoremediation, as well as on the entire ecosystem (Kumpiene et al., 2008; Vangronsveld et al., 2009; Wiszniewska et al., 2016; DalCorso et al., 2019).
Plant Genetic Breeding for Phytoremediation Applications
The ability of plants to take up, translocate, and transform trace elements, as well as to limit their toxicity, may be significantly enhanced via genetic engineering (for a comprehensive review see Koźmińska et al., 2018). Although genetic transformation has been developed or improved for several grass species during the last decades (Wang and Ge, 2006), this technology is still poorly explored in the context of trace element phytoremediation. Only a few studies employed genetic manipulation to improve the desirable characteristics of grasses for trace element phytoremediation (Czakó et al., 2005, 2006; Taghizadeh et al., 2015).
Czakó et al. (2005) assessed the transfer of gene encoding enzymes for the breaking down of organomercurials [Organomercurial lyase (MerB, EC 4.99.1.2)] and the reduction of Hg2+ to Hg0 [Mercuric reductase (MerA) (EC 1.16.1.1)] into the S. alterniflora. They reported that all but one transgenic line contained both MerB and MerA sequences, demonstrating that the co-introduction into S. alterniflora of two genes from separate Agrobacterium strains is possible. A wild-type callus of S. alterniflora was sensitive to phenylmercuric acetate (PMA) at 50 μmol L–1 and to HgCl2 at 225 μmol L–1, but the transgenic line #3 was resistant to PMA, whereas the transgenic line #7 showed resistance to HgCl2 (up to 500 μmol L–1) (Czakó et al., 2006). Plant breeding by in vitro culture to improve the tolerance and accumulation of Pb in C. dactylon was evaluated and the results of the study revealed the occurrence of somaclonal variation via somatic embryogenesis and organogenesis of C. dactylon cultures with a frequency of 33% (Taghizadeh et al., 2015). Some in vitro derived plants had higher Pb concentrations in the shoot, but there were also some regenerates with lower Pb concentrations in the shoot and some without any changes in Pb concentrations compared with the control.
Although some progress has been achieved after a long-term in vitro selection of trace element-tolerant clones during the last years, modifications involving genome editing and genome engineering to insert gene(s) responsible for trace element transport and homeostasis or defense responses to oxidative stress can be a more efficient strategy (Koźmińska et al., 2018). Numerous pathways can be manipulated to increase the trace elements uptake, translocation, and accumulation in plant tissues. However, to increase trace elements uptake by grasses it is necessary to increase their tolerance, otherwise, the phytotoxicity induced by trace elements certainly will lead to the death of the plant. The genes that are currently widely used to manipulate trace element metabolism in plants are those that encode for transporters of trace element ions and trace element-binding ligands (e.g., GSH1 and PCS) (Yadav, 2010; Koźmińska et al., 2018). However, to the best of our knowledge, there is no study about this in grasses used for phytoremediation.
Advantages and Disadvantages of the Use of Grasses for Trace Element Phytoremediation
The use of grasses for trace element phytoremediation has advantages and disadvantages compared with the use of other plant species; some particularities are described below.
Advantages
(i) Grasses colonize land from cold regions to humid wetlands in the tropics spontaneously (Zeven and de Wet, 1982) and can be efficiently used for phytoextraction of mildly polluted agricultural soils (Figure 5), phytostabilization, or phytofiltration of trace elements (Table 2) during the rehabilitation of polluted areas (Figure 6);
(ii) Perennial grasses exposed to trace elements can sprout again after shoot harvest (Gilabel et al., 2014; Rabêlo et al., 2017a), avoiding the need of replanting after each harvest;
(iii) Grasses often deliver high biomass yield (sometimes more than 20 t DW ha–1 yr–1; Rabêlo et al., 2018a) that can be used after harvesting as a source for bioenergy (biogas, bioethanol, bio-oil) (Balsamo et al., 2015), thus with added economical value and eventually, also for the recovery of trace elements;
(iv) Growing and harvesting grasses do not require expensive equipment or highly qualified staff.
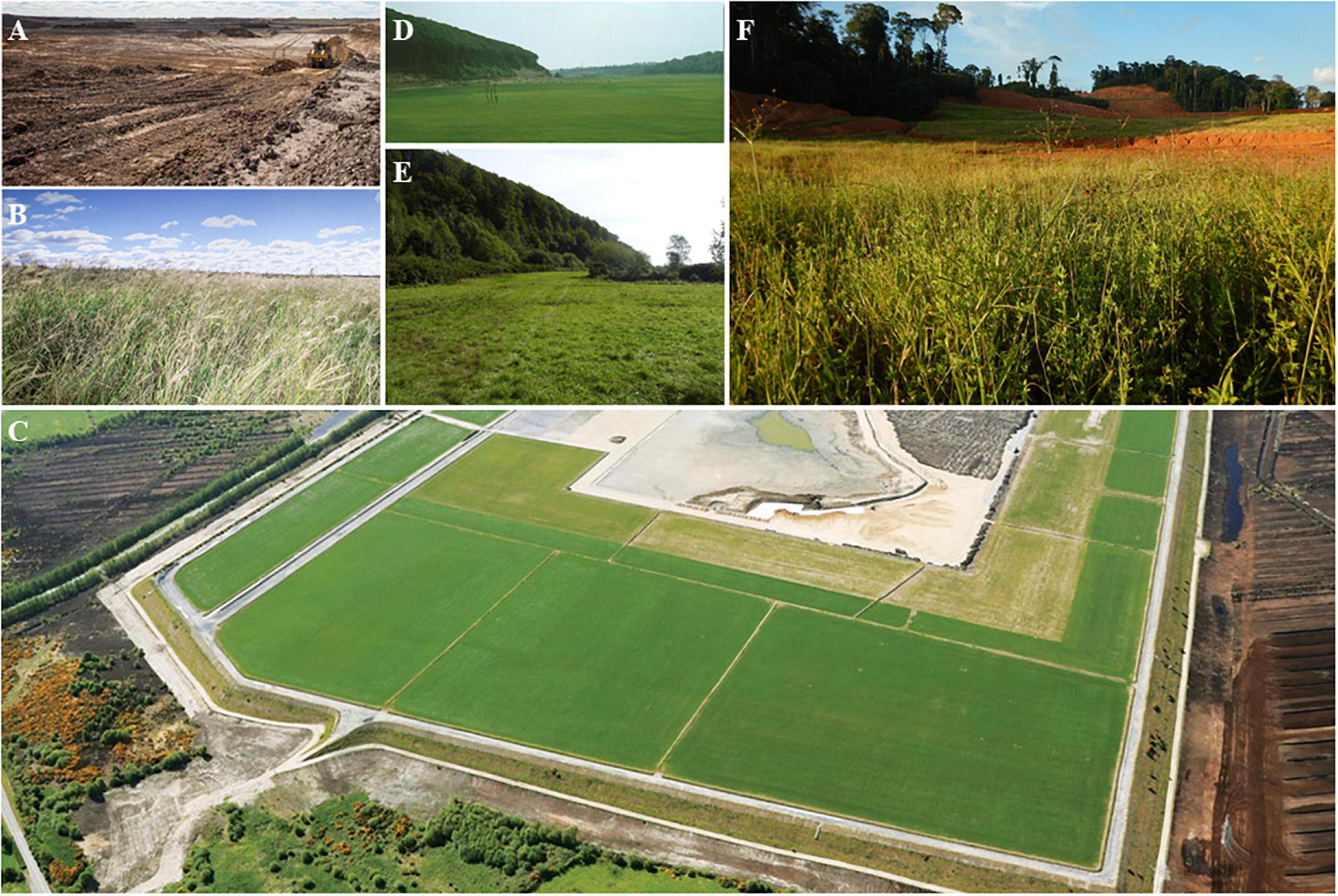
Figure 6. Rehabilitation of mining areas in Australia (A,B), Ireland (C–E), and Guyana (F) by using grasses (grass species not specified). A: Commodore Coal Mine before rehabilitation (Minerals Council of Australia [MCA], 2016); B: Commodore Coal Mine after rehabilitation (Minerals Council of Australia [MCA], 2016); C: Tailings management facility rehabilitation at Lisheen Mine (Courtney, 2018); D: Shelton Abbey rehabilitated tailings site after shortly seeding in 1982 (Courtney, 2018); E: Shelton Abbey rehabilitated tailings site after seeding in September 2016 (Courtney, 2018); F: Rehabilitation of mining sites located in the heart of the Amazon rainforest (https://esperancegoldmine.com/index.php/en/rehabilitation-of-mine-sites/).
Disadvantages
(i) The growth and biomass yield of grasses (mainly of those grasses with high biomass yield) can slow down or even stop due to water deficit (Ings et al., 2013) and low temperatures (Lewandowski et al., 2003), which compromises the phytoremediation efficiency;
(ii) The use of grasses for phytoremediation is limited to the upper soil layer. The rooting depth varies with the grass species used, but often a major fraction of the root system is concentrated in the upper 0–15 cm (Gist and Smith, 1948);
(iii) Many grass species can only be used in sites polluted with one or two trace elements, which restricts their use in multi-element polluted sites (Table 2).
General Conclusion and Perspectives
Undoubtedly, grasses have a tremendous potential to stabilize trace elements in soils, sediments, and wastewater. However, much more studies employing molecular approaches are required to better understand the processes involved in the uptake, transport, and accumulation of trace elements. Several types of grass have been investigated, but only a limited number of transporters of As, Cd, Cu, and Zn have been identified in just a few species. The expression of genes encoding for trace element transporters is species- and tissue-specific. Understanding the role of each trace element transporter in grasses is essential to optimize the phytoremediation of trace elements. Studies aiming to identify trace element transporters and to elucidate mechanisms related to uptake, transport, and accumulation of trace elements should be conducted, especially for Cr, Hg, Ni, and Pb. The most existing studies aiming to better understand the physiological processes of the induction of trace element toxicity and how grasses cope with this toxicity are based on acute trace elements exposure. This makes it difficult to link the results to tolerance mechanisms since, in the first stage of trace element exposure, a sequence of signaling events occurs to adapt the plant to the stress. Under field conditions, however, exposure to a trace element is chronic which often provokes a range of responses very different from those observed in case of acute exposure (Gallego et al., 2012). Therefore, studies need to be conducted under more realistic scenarios, since most grasses do not survive for a long time when exposed to high doses of different trace elements. A range of grass species may be suitable for the phytoextraction of mildly polluted agricultural soils or phytostabilization of soils with low-to-moderate concentrations of trace elements, and only a few species are appropriate for phytofiltration of trace elements. The efficacies of grasses for phytoextraction or phytostabilization can be improved using amendments and microorganisms supporting plant growth, but the adoption of such strategies should be assessed case-by-case. Increases in the trace element uptake by plants instead of better trace element phytostabilization can occur and vice versa due to the specificity of trace elements, amendment/microorganisms, and plant interactions. Genetic manipulation, which is another alternative to increase the efficiencies of phytoextraction and phytostabilization of trace elements, has been largely neglected due to its genetic complexity and the associated difficulties encountered during conventional breeding of grasses (Wang and Ge, 2006), leaving an important gap open that might be explored during the next years. The rehabilitation of trace element-polluted sites by using only grasses was proven to be an efficient strategy (Minerals Council of Australia [MCA], 2016; Courtney, 2018), but the cultivation of grasses associated with other plants such as legumes and trees can accelerate site rehabilitation and is more adequate from an ecological point of view (Kumar et al., 2017; Wen et al., 2018).
Author Contributions
FHSR conceived the project and wrote the manuscript. JV, AJMB, AVDE, and LRFA reviewed the manuscript by rewriting, discussing, and commenting. All the authors contributed to the article and approved the submitted version.
Funding
This review was supported through financial support for FHSR by the Brazilian Federal Government Agency, Coordination for the Improvement of Higher Education Personnel - CAPES (grant number 1332394), by the National Council for Scientific and Technological Development CNPq (grant number 306403/2013-7), and by the São Paulo Research Foundation – FAPESP (grants number 2012/12894-3, 2012/11859-0, 2014/16731-7, 2014/18735-0, 2015/21562-2, 2017/11299-8, and 2018/07190-3).
Conflict of Interest
The authors declare that the research was conducted in the absence of any commercial or financial relationships that could be construed as a potential conflict of interest.
Publisher’s Note
All claims expressed in this article are solely those of the authors and do not necessarily represent those of their affiliated organizations, or those of the publisher, the editors and the reviewers. Any product that may be evaluated in this article, or claim that may be made by its manufacturer, is not guaranteed or endorsed by the publisher.
References
Abbas, G., Murtaza, B., Bibi, I., Shahid, M., Niazi, N. K., Khan, M. I., et al. (2018). Arsenic uptake, toxicity, detoxification, and speciation in plants: physiological, biochemical, and molecular aspects. Int. J. Environ. Res. Public Health 15:59. doi: 10.3390/ijerph15010059
Aboulroos, S. A., Helal, M. I. D., and Kamel, M. M. (2006). Remediation of Pb and Cd polluted soils using in situ immobilization and phytoextraction techniques. Soil. Sediment. Contam. 15, 199–215. doi: 10.1080/15320380500506362
Adabnejad, H., Kavousi, H. R., Hamidi, H., and Tavassolian, I. (2015). Assessment of the vacuolar Na+ /H+ antiporter (NHX1) transcriptional changes in Leptochloa fusca L. in response to salt and cadmium stresses. Mol. Biol. Res. Commun. 4, 133–142.
Alagarasan, G., Dubey, M., Aswathy, K. S., and Chandel, G. (2017). Genome wide identification of orthologous ZIP genes associated with zinc and iron translocation in Setaria italica. Front. Plant Sci. 8:775. doi: 10.3389/fpls.2017.00775
Albornoz, C. B., Larsen, K., Landa, R., Quiroga, M. A., Najle, R., and Marcovecchio, J. (2016). Lead and zinc determinations in Festuca arundinacea and Cynodon dactylon collected from contaminated soils in Tandil (Buenos Aires Province, Argentina). Environ. Earth. Sci. 75:742. doi: 10.1007/s12665-016-5513-9
Alkorta, I., Becerril, J. M., and Garbisu, C. (2010). Phytostabilization of metal contaminated soils. Environ. Health Rev. 25, 135–146. doi: 10.1515/REVEH.2010.25.2.135
Amin, H., Arain, B. A., Abbasi, M. S., Amin, F., Jahangir, T. M., and Soomro, N. (2019). Evaluation of chromium phyto-toxicity, phyto-tolerance, and phyto-accumulation using biofuel plants for effective phytoremediation. Int. J. Phytoremediat. 21, 352–363. doi: 10.1080/15226514.2018.1524837
Andra, S. S., Datta, R., Sarkar, D., Makris, K. C., Mullens, C. P., Sahi, S. V., et al. (2010). Synthesis of phytochelatins in vetiver grass upon lead exposure in the presence of phosphorus. Plant Soil 326, 171–185. doi: 10.1007/s11104-009-9992-2
Andrade, A. F. M., Amaral Sobrinho, N. M. B., Santos, F. S., Magalhães, M. O. L., Tolón-Becerra, A., and Lima, L. S. (2014). EDTA-induced phytoextraction of lead and barium by brachiaria (B. decumbens cv. Basilisk) in soil contaminated by oil exploration drilling waste. Acta Sci.-Agron. 36, 495–500. doi: 10.4025/actasciagron.v36i4.18172
Andresen, E., Peiter, E., and Küpper, H. (2018). Trace metal metabolism in plants. J. Exp. Bot. 69, 909–954. doi: 10.1093/jxb/erx465
Anjum, N. A., Ahmad, I., Rodrigues, S. M., Henriques, B., Cruz, N., Coelho, C., et al. (2013). Eriophorum angustifolium and Lolium perenne metabolic adaptations to metals- and metalloids-induced anomalies in the vicinity of a chemical industrial complex. Environ. Sci. Pollut. Res. 20, 568–581. doi: 10.1007/s11356-012-1062-2
Antiochia, R., Campanella, L., Ghezzi, P., and Movassaghi, K. (2007). The use of vetiver for remediation of heavy metal soil contamination. Anal. Bioanal. Chem. 388, 947–956. doi: 10.1007/s00216-007-1268-1
Araújo, A. S. A., Guilherme, L. R. G., Lopes, G., and Campos, M. L. (2011). Phytoremediation of arsenic-contaminated soils using Brachiaria grass. Cienc. Agrotec. 35, 84–91. doi: 10.1590/S1413-70542011000100010
Arroyave, Q. C., Araque, M. P., and Pelaez, J. C. A. (2010). Bioaccumulation and toxicity evaluation of cadmium and mercury in Llanero grass (Brachiaria dictyoneura). Vitae 17, 45–49.
Awa, S. H., and Hadibarata, T. (2020). Removal of heavy metals in contaminated soil by phytoremediation mechanism: a review. Water Air Soil Pollut. 23:47. doi: 10.1007/s11270-020-4426-0
Azeez, J. O., Olowoboko, T. B., Bada, B. S., Odedina, J. N., and Onasanya, O. O. (2020). Evaluation of soil metal sorption characteristics and heavy metal extractive ability of indigenous plant species in Abeokuta. Nigeria. Int. J. Phytoremediat. 22, 872–884. doi: 10.1080/15226514.2020.1717433
Baker, A. J. M. (1981). Accumulators and excluders - strategies in the response of plants to heavy metals. J. Plant Nutr. 3, 643–654. doi: 10.1080/01904168109362867
Balsamo, R. A., Kelly, W. J., Satrio, J. A., Ruiz-Felix, M. N., Fetterman, M., Wynn, R., et al. (2015). Utilization of grasses for potential biofuel production and phytoremediation of heavy metal contaminated soils. Int. J. Phytoremediat. 17, 448–455. doi: 10.1080/15226514.2014.922918
Becerra-Castro, C., Monterroso, C., Prieto-Fernández, A., Rodríguez-Lamas, L., Loureiro-Viñas, M., Acea, M. J., et al. (2012). Pseudometallophytes colonising Pb/Zn mine tailings: a description of the plant-microorganism-rhizosphere soil system and isolation of metal-tolerant bacteria. J. Hazard. Mater. 21, 350–359. doi: 10.1016/j.jhazmat.2012.03.039
Boros-Lajszner, E., Wyszkowska, J., and Kucharski, J. (2021). Phytoremediation of soil contaminated with nickel, cadmium and cobalt. Int. J. Phytoremediat. 23, 252–262. doi: 10.1080/15226514.2020.1807907
Broadley, M. R., White, P. J., Hammond, J. P., Zelko, I., and Lux, A. (2007). Zinc in plants. New Phytol. 173, 677–702. doi: 10.1111/j.1469-8137.2007.01996.x
Busby, R. R., Douglas, T. A., LeMonte, J. J., Ringelberg, D. B., and Indest, K. J. (2020). Metal accumulation capacity in indigenous Alaska vegetation growing on military training lands. Int. J. Phytoremediat. 22, 259–266. doi: 10.1080/15226514.2019.1658708
Buscaroli, A. (2017). An overview of indexes to evaluate terrestrial plants for phytoremediation purposes (Review). Ecol. Indic. 82, 367–380. doi: 10.1016/j.ecolind.2017.07.003
Cakmak, I., Öztürk, L., and Karanlik, S. (1996). Zinc-efficient wild grasses enhance release of phytosiderophores under zinc deficiency. J. Plant Nutr. 19, 551–563. doi: 10.1080/01904169609365142
Carbonell-Barrachina, A., Aarabi, M., DeLaune, R., Gambrell, R. P., and Patrick, W. H. Jr. (1998). The influence of arsenic chemical form and concentration on Spartina patens and Spartina alterniflora growth and tissue arsenic concentration. Plant Soil 198, 33–43. doi: 10.1023/A:1004285625998
Chai, M. W., Li, R. L., Shi, F. C., Liu, F. C., Pan, X., Cao, D., et al. (2012). Effects of cadmium stress on growth, metal accumulation and organic acids of Spartina alterniflora Loisel. Afr. J. Biotechnol. 11, 6091–6099. doi: 10.5897/AJB11.2804
Chandra, R., Kumar, V., Tripathi, S., and Sharma, P. (2018). Heavy metal phytoextraction potential of native weeds and grasses from endocrine-disrupting chemicals rich complex distillery sludge and their histological observations during in-situ phytoremediation. Ecol. Eng. 111, 143–156. doi: 10.1016/j.ecoleng.2017.12.007
Chaney, R. L., Malik, M., Li, Y. M., Brown, S. L., Brewer, E. P., Angle, J. S., et al. (1997). Phytoremediation of soil metals. Curr. Opin. Biotechnol. 8, 279–284. doi: 10.1016/S0958-1669(97)80004-3
Chen, J., and Yang, Z. M. (2012). Mercury toxicity, molecular response and tolerance in higher plants. Biometals 25, 847–857. doi: 10.1007/s10534-012-9560-8
Chen, Q., and Wong, J. W. C. (2006). Growth of Agropyron elongatum in a simulated nickel contaminated soil with lime stabilization. Sci. Total Environ. 366, 448–455. doi: 10.1016/j.scitotenv.2005.01.022
Chen, Y.-T., Wang, Y., and Yeh, K.-C. (2017). Role of root exudates in metal acquisition and tolerance. Curr. Opin. Plant Biol. 39, 66–72. doi: 10.1016/j.pbi.2017.06.004
Chintani, Y. S., Butarbutar, E. S., Nugroho, A. P., and Sembiring, T. (2021). Uptake and release of chromium and nickel by Vetiver grass (Chrysopogon zizanioides (L.) Roberty). SN Appl. Sci. 3:285. doi: 10.1007/s42452-021-04298-w
Clemens, S. (2006). Toxic metal accumulation, responses to exposure and mechanisms of tolerance in plants. Biochimie 88, 1707–1719. doi: 10.1016/j.biochi.2006.07.003
Clemens, S., and Ma, J. F. (2016). Toxic heavy metal and metalloid accumulation in crop plants and foods. Annu. Rev. Plant Biol. 67, 12.1–12.24. doi: 10.1146/annurev-arplant-043015-112301
Cobbett, C., and Goldsbrough, P. (2002). Phytochelatins and metallothioneins: roles in heavy metal detoxification and homeostasis. Annu. Rev. Plant Biol. 53, 159–182. doi: 10.1146/annurev.arplant.53.100301.135154
Conesa, H. M., and Faz, A. (2011). Metal uptake by spontaneous vegetation in acidic mine tailings from a Semiarid area in South Spain: implications for revegetation and land management. Water Air Soil Pollut. 215, 221–227. doi: 10.1007/s11270-010-0471-4
Courtney, R. (2018). “Irish mine sites rehabilitation - A case study,” in Bio-Geotechnologies for Mine Site Rehabilitation, eds M. N. V. Prasad, P. J. C. Favas, and S. K. Maiti (Amsterdam: Elsevier), 439–456.
Cuypers, A., Hendrix, S., dos Reis, R., De Smet, S., Deckers, J., Gielen, H., et al. (2016). Hydrogen peroxide, signaling in disguise during metal phytotoxicity. Front. Plant Sci. 7:470. doi: 10.3389/fpls.2016.00470
Czakó, M., Feng, X., He, Y., Liang, D., and Márton, L. (2005). Genetic modification of wetland grasses for phytoremediation. Z. Naturforsch. (C) 60, 285–291. doi: 10.1515/znc-2005-3-414
Czakó, M., Feng, X., He, Y., Liang, D., and Márton, L. (2006). Transgenic Spartina alterniflora for phytoremediation. Environ. Geochem. Health 28, 103–110. doi: 10.1007/s10653-005-9019-8
DalCorso, G., Fasani, E., Manara, A., Visioli, G., and Furini, A. (2019). Heavy metal pollutions: state of the art and innovation in phytoremediation. Int. J. Mol. Sci. 20:3412. doi: 10.3390/ijms20143412
Darabi, S. A. S., Almodares, A., and Ebrahim, M. (2017). In silico study shows arsenic induces P1B ATPase gene family as cation transporter by abscisic acid signaling pathway in seedling of Sorghum bicolor. Acta Physiol. Plant 39:172. doi: 10.1007/s11738-017-2472-z
De Conti, L., Marques, A. C. R., Ceretta, C. A., Tarouco, C. P., Nicoloso, F. T., Ferreira, F. T., et al. (2020). Tolerance and phytoremediation potential of grass species native to South American grasslands to copper-contaminated soils. Int. J. Phytoremediat. 30, 1–10. doi: 10.1080/15226514.2020.1852528
de Sousa Leite, T., and Monteiro, F. A. (2019a). Nitrogen form regulates cadmium uptake and accumulation in Tanzania guinea grass used for phytoextraction. Chemosphere 236:124324. doi: 10.1016/j.chemosphere.2019.07.055
de Sousa Leite, T., and Monteiro, F. A. (2019b). Partial replacement of nitrate by ammonium increases photosynthesis and reduces oxidative stress in tanzania guinea grass exposed to cadmium. Ecotox. Environ. Safe. 174, 592–600. doi: 10.1016/j.ecoenv.2019.02.090
De Temmerman, L., Claey, N., Roekens, E., and Guns, M. (2007). Biomonitoring of airborne mercury with perennial ryegrass cultures. Environ. Pollut. 146, 458–462. doi: 10.1016/j.envpol.2006.07.002
Deng, H., Ye, Z. H., and Wong, M. H. (2006). Lead and zinc accumulation and tolerance in populations of six wetland plants. Environ. Pollut. 141, 69–80. doi: 10.1016/j.envpol.2005.08.015
Dhankher, O. P., Li, Y. J., Rosen, B. P., Shi, J., Salt, D., Senecoff, J. F., et al. (2002). Engineering tolerance and hyperaccumulation of arsenic in plants by combining arsenate reductase and gammaglutamylcysteine synthetase expression. Nat. Biotechnol. 20, 1140–1145. doi: 10.1038/nbt747
Ding, W., Zhang, J., Wu, S.-C., Zhang, S., Christie, P., and Liang, P. (2019). Responses of the grass Paspalum distichum L. to Hg stress: a proteomic study. Ecotox. Environ. Safe. 183:109549. doi: 10.1016/j.ecoenv.2019.109549
Dradach, A., Karczewska, A., and Szopka, K. (2020b). Arsenic uptake by two tolerant grass species: holcus lanatus and Agrostis capillaris growing in soils contaminated by historical mining. Plants 9:980. doi: 10.3390/plants9080980
Dradach, A., Karczewska, A., and Szopka, K. (2020a). Arsenic accumulation by red fescue (Festuca rubra) growing in mine affected soils - Findings from the field and greenhouse studies. Chemosphere 248:126045. doi: 10.1016/j.chemosphere.2020.126045
Ebbs, S. D., Lasat, M. M., Brady, D. J., Cornish, J., Gordon, R., and Kochian, L. V. (1997). Phytoextraction of cadmium and zinc from a contaminated soil. J. Environ. Qual. 26, 1424–1430. doi: 10.2134/jeq1997.00472425002600050032x
El-Gendy, A. S., El-Kassas, H. I., Razek, T. M. A., and Abdel-Latif, H. (2017). Phyto-dewatering of sewage sludge using Panicum repens. Water Sci. Technol. 75, 1667–1674. doi: 10.2166/wst.2017.039
Elhawat, N., Alshaal, T., Domokos-Szabolcsy, E., El-Ramady, H., Antal, G., Márton, L., et al. (2015). Copper uptake efficiency and its distribution within bioenergy grass Giant Reed. Bull. Environ. Contam. Toxicol. 95, 452–458. doi: 10.1007/s00128-015-1622-5
Ertani, A., Mietto, A., Borin, M., and Nardi, S. (2017). Chromium in agricultural soils and crops: a review. Water Air Soil Pollut. 228:190. doi: 10.1007/s11270-017-3356-y
Esteban, E., Moreno, E., Peñalosa, J., Cabrero, J. I., Millán, R., and Zornoza, P. (2008). Short and long-term uptake of Hg in white lupin plants: kinetics and stress indicators. Environ. Exp. Bot. 62, 316–322. doi: 10.1016/j.envexpbot.2007.10.006
Fasani, E., DalCorso, G., Zerminiani, A., Ferrarese, A., Campostrini, P., and Furini, A. (2019). Phytoremediatory efficiency of Chrysopogon zizanioides in the treatment of landfill leachate: a case study. Environ. Sci. Pollut. Res. 26, 10057–10069. doi: 10.1007/s11356-019-04505-7
Fernández, S., Poschenrieder, C., Marcenò, C., Gallego, J. R., Jiménez-Gámez, D., Bueno, A., et al. (2017). Phytoremediation capability of native plant species living on Pb-Zn and Hg-As mining wastes in the Cantabrian range, north of Spain. J. Geochem. Explor. 174, 10–20. doi: 10.1016/j.gexplo.2016.05.015
Fernández-Llamosas, H., Ibero, J., Thijs, S., Imperato, V., Vangronsveld, J., Díaz, E., et al. (2020). Enhancing the rice seedlings growth promotion abilities of Azoarcus sp. CIB by heterologous expression of ACC deaminase to improve performance of plants exposed to cadmium stress. Microorganisms 8:1453. doi: 10.3390/microorganisms8091453
Fitamo, D., Leta, S., Belay, G., Lemma, B., and Olsson, M. (2011). Phytoavailability of heavy metals and metalloids in soils irrigated with wastewater, Akaki, Ethiopia: a Greenhouse Study. Soil Sediment. Contam. 20, 745–766. doi: 10.1080/15320383.2011.609196
Food and Agriculture Organization of the United Nations and the United Nations Environment Programme (2021). Global Assessment of Soil Pollution – Summary for Policy Makers. Rome: FAO.
Freitas, M. C., Pacheco, A. M. G., Anawar, H. M., Dionísio, I., Dung, M., Canha, N., et al. (2009). Determination of phytoextraction potential of plant species for toxic elements in soils of abandoned sulphide-mining areas. J. Radioanal. Nucl. Chem. 282, 21–27. doi: 10.1007/s10967-009-0222-4
Fu, S., Wei, C., and Li, L. (2016). Characterizing the accumulation of various heavy metals in native plants growing around an old antimony mine. Hum. Ecol. Risk Assess. 22, 882–898. doi: 10.1080/10807039.2015.1118676
Gajaje, K., Ultra, V. U., David, P. W., and Rantong, G. (2021). Rhizosphere properties and heavy metal accumulation of plants growing in the fly ash dumpsite, Morupule power plant, Botswana. Environ. Sci. Pollut. Res Int. 28, 20637–20649. doi: 10.1007/s11356-020-11905-7
Gallego, S. M., Pena, L. B., Barcia, R. A., Azpilicueta, C. E., Iannone, M. F., Rosales, E. P., et al. (2012). Unravelling cadmium toxicity and tolerance in plants: insight into regulatory mechanisms. Environ. Exp. Bot. 83, 33–46. doi: 10.1016/j.envexpbot.2012.04.006
Gao, B., Zhang, X., Tian, C., Zhang, X., and Liu, J. (2020). Effects of amendments and aided phytostabilization of an energy crop on the metal availability and leaching in mine tailings using a pot test. Environ. Sci. Pollut. Res. 27, 2745–2759. doi: 10.1007/s11356-019-07171-x
Gilabel, A. P., Nogueirol, R. C., Garbo, A. I., and Monteiro, F. A. (2014). The role of sulfur in increasing guinea grass tolerance of copper phytotoxicity. Water Air Soil Pollut. 225:1806. doi: 10.1007/s11270-013-1806-8
Gist, G. R., and Smith, R. M. (1948). Root development of several common forage grasses to a depth of eighteen inches. Agron. J. 40, 1036–1042. doi: 10.2134/agronj1948.00021962004000110008x
Gomes, M. P., Sá e Melo Marques, T. C. L. L., Nogueiroa, M. O. G., Castro, E. M., and Soares, A. M. (2011). Ecophysiological and anatomical changes due to uptake and accumulation of heavy metal in Brachiaria decumbens. Sci. Agric. 68, 566–573. doi: 10.1590/S0103-90162011000500009
Gomes, P., Valente, T., Pamplona, J., Braga, M. A. S., Pissarra, J., Gil, J. A. G., et al. (2014). Metal uptake by native plants and revegetation potential of mining sulfide-rich waste-dumps. Int. J. Phytoremediat. 16, 1087–1103. doi: 10.1080/15226514.2013.810586
Gómez, J., Yunta, F., Esteban, E., Carpena, R. O., and Zornoza, P. (2016). Use of radiometric indices to evaluate Zn and Pb stress in two grass species (Festuca rubra L. and Vulpia myuros L.). Environ. Sci. Pollut. Res. 23, 23239–23248. doi: 10.1007/s11356-016-7546-8
Gratão, P. L., Polle, A., Lea, P. J., and Azevedo, R. A. (2005). Making the life of heavy metal-stressed plants a little easier. Funct. Plant Biol. 32, 481–494. doi: 10.1071/FP05016
Guo, Q., Meng, L., Humphreys, M. W., Scullion, J., and Mur, L. A. J. (2017). Expression of FlHMA3, a P1B2-ATPase from Festulolium loliaceum, correlates with response to cadmium stress. Plant Physiol. Biochem. 112, 270–277. doi: 10.1016/j.plaphy.2017.01.013
Gutiérrez-Ginés, M. J., Pastor, J., and Hernández, A. J. (2012). Integrated approach to assessing the effects of soils polluted with heavy metals on a plant population. Ecotoxicology 21, 1965–1978. doi: 10.1007/s10646-012-0931-2
Hall, J. L. (2002). Cellular mechanisms for heavy metal detoxification and tolerance. J. Exp. Bot. 53, 1–11. doi: 10.1093/jexbot/53.366.1
Harrington, C. F., Roberts, D. J., and Nlckless, G. (1996). The effect of cadmium, zinc, and copper on the growth, tolerance index, metal uptake, and production of malic acid in two strains of the grass Festuca rubra. Can. J. Bot. 74, 1742–1752. doi: 10.1139/b96-211
Hayward, A. R., Coates, K. E., Galer, A. L., Hutchinson, T. C., and Emery, R. J. N. (2013). Chelator profiling in Deschampsia cespitosa (L.) Beauv. Reveals a Ni reaction, which is distinct from the ABA and cytokinin associated response to Cd. Plant Physiol. Biochem. 64, 84–91. doi: 10.1016/j.plaphy.2012.12.018
He, Z., Shentu, J., Yang, X., Baligar, V. C., Zhang, T., and Stoffella, P. J. (2015). Heavy metal contamination of soils: sources, indicators, and assessment. J. Environ. Indic. 9, 17–18.
Hesami, R., Salimi, A., and Ghaderian, S. M. (2018). Lead, zinc, and cadmium uptake, accumulation, and phytoremediation by plants growing around Tang-e Douzan lead-zinc mine, Iran. Environ. Sci. Pollut. Res. 25, 8701–8714. doi: 10.1007/s11356-017-1156-y
Hodson, M. J., White, P. J., Mead, A., and Broadley, M. R. (2005). Phylogenetic variation in the silicon composition of plants. Ann. Bot. 96, 1027–1046. doi: 10.1093/aob/mci255
Hoehne, L., de Lima, C. V. S., Martini, M. C., Altmayer, T., Brietzke, D. T., Finatto, J., et al. (2016). Addition of vermicompost to heavy metal-contaminated soil increases the ability of Black Oat (Avena strigosa Schreb) plants to remove Cd, Cr, and Pb. Water Air Soil Pollut. 227:443. doi: 10.1007/s11270-016-3142-2
Hou, X., Teng, W., Hu, Y., Yang, Z., Li, C., Scullion, J., et al. (2020). Potential phytoremediation of soil cadmium and zinc by diverse ornamental and energy grasses. BioResources 15, 616–640.
Ings, J., Mur, L. A. J., Robson, P. R. H., and Bosch, M. (2013). Physiological and growth responses to water deficit in the bioenergy crop Miscanthus × giganteus. Front. Plant Sci. 4:468. doi: 10.3389/fpls.2013.00468
Jacobs, A., Drouet, T., and Noret, N. (2018). Field evaluation of cultural cycles for improved cadmium and zinc phytoextraction with Noccaea caerulescens. Plant Soil 430, 381–394. doi: 10.1007/s11104-018-3734-2
Jiang, X., and Wang, C. (2008). Zinc distribution and zinc-binding forms in Phragmites australis under zinc pollution. J. Plant Physiol. 165, 697–704. doi: 10.1016/j.jplph.2007.05.011
Jung, H.-I., Gayomba, S. R., Yan, J., and Vatamaniuk, O. K. (2014). Brachypodium distachyon as a model system for studies of copper transport in cereal crops. Front. Plant Sci. 5:236. doi: 10.3389/fpls.2014.00236
Kayser, A., Wenger, K., Keller, A., Attinger, W., Felix, H. R., Gupta, S. K., et al. (2000). Enhancement of phytoextraction of Zn, Cd, and Cu from calcareous soil: the use of NTA and sulfur amendments. Environ. Sci. Technol. 34, 1778–1783. doi: 10.1021/es990697s
Kaznina, N. M., and Titov, A. F. (2014). The influence of cadmium on physiological processes and productivity of Poaceae plants. Biol. Bull. Rev. 4, 335–348. doi: 10.1134/S2079086414040057
Khalid, B. Y., and Tinsley, J. (1980). Some effects of nickel toxicity on Rye grass. Plant Soil 55, 139–144. doi: 10.1007/BF02149717
Khan, M. M., Islam, E., Irem, S., Akhtar, K., Ashraf, M. Y., Iqbal, J., et al. (2018). Pb-induced phytotoxicity in para grass (Brachiaria mutica) and Castorbean (Ricinus communis L.): antioxidant and ultrastructural studies. Chemosphere 200, 257–265. doi: 10.1016/j.chemosphere.2018.02.101
Kim, S., Lim, H., and Lee, I. (2010). Enhanced heavy metal phytoextraction by Echinochloa crus-galli using root exudates. J. Biosci. Bioeng. 109, 47–50. doi: 10.1016/j.jbiosc.2009.06.018
Kitczak, T., Kiepas-Kokot, A., and Czyż, H. (2016). Yielding and heavy metals accumulation in the biomass of grass cultivated in substrata with the participation of municipal sewage sludge and green waste compost. Pol. J. Environ. Stud. 25, 2009–2014. doi: 10.15244/pjoes/62796
Kocoń, A., and Jurga, B. (2017). The evaluation of growth and phytoextraction potential of Miscanthus x giganteus and Sida hermaphrodita on soil contaminated simultaneously with Cd, Cu, Ni, Pb, and Zn. Environ. Sci. Pollut. Res. 24, 4990–5000. doi: 10.1007/s11356-016-8241-5
Kopittke, P. M., Asher, C. J., Blamey, F. P., Auchterlonie, G. J., Guo, Y. N., and Menzies, N. W. (2008). Localization and chemical speciation of Pb in roots of signal grass (Brachiaria decumbens) and Rhodes grass (Chloris gayana). Environ. Sci. Technol. 42, 4595–4599. doi: 10.1021/es702627c
Kopittke, P. M., Blamey, F. P. C., Kopittke, R. A., Asher, C. J., and Menzies, N. W. (2010). Tolerance of seven perennial grasses to high nickel in sand culture. Environ. Chem. 7, 279–286. doi: 10.1071/EN09100
Kopittke, P. M., Menzies, N. W., de Jonge, M. D., McKenna, B. A., Donner, E., Webb, R. I., et al. (2011). In situ distribution and speciation of toxic copper, nickel, and zinc in hydrated roots of Cowpea. Plant Physiol. 156, 663–673. doi: 10.1104/pp.111.173716
Korzeniowska, J., and Stanislawska-Glubiak, E. (2015). Phytoremediation potential of Miscanthus × giganteus and Spartina pectinata in soil contaminated with heavy metals. Environ. Sci. Pollut. Res. 22, 11648–11657. doi: 10.1007/s11356-015-4439-1
Korzeniowska, J., and Stanislawska-Glubiak, E. (2019). Phytoremediation potential of Phalaris arundinacea, Salix viminalis and Zea mays for nickel-contaminated soils. Int. J. Environ. Sci. Technol. 16, 1999–2008. doi: 10.1007/s13762-018-1823-7
Korzeniowska, J., Stanisławska-Glubiak, E., and Igras, J. (2011). Applicability of energy crops for metal phytostabilization on lands moderately contaminated with Copper, Nickel and Zinc. J. Food Agric. Environ. 9, 693–697.
Kowitwiwat, A., and Sampanpanish, P. (2020). Phytostabilization of arsenic and manganese in mine tailings using Pennisetum purpureum cv. Mott supplemented with cow manure and acacia wood-derived biochar. Heliyon 6:e04552. doi: 10.1016/j.heliyon.2020.e04552
Koźmińska, A., Wiszniewska, A., Hanus-Fajerska, E., and Muszyńska, E. (2018). Recent strategies of increasing metal tolerance and phytoremediation potential using genetic transformation of plants. Plant Biotechnol. Rep. 12, 1–14. doi: 10.1007/s11816-017-0467-2
Kristanti, A., Ngu, W. J., Yuniarto, A., and Hadibarata, T. (2021). Rhizofiltration for removal of inorganic and organic pollutants in groundwater: a review. Biointerface Res. Appl. Chem. 11, 12326–12347. doi: 10.33263/BRIAC114.1232612347
Krzesłowska, M. (2011). The cell wall in plant cell response to trace metals: polysaccharide remodeling and its role in defense strategy. Acta Physiol. Plant. 33, 35–51. doi: 10.1007/s11738-010-0581-z
Kullu, B., Patra, D. K., Acharya, S., Pradhan, C., and Patra, H. K. (2020). AM fungi mediated bioaccumulation of hexavalent chromium in Brachiaria mutica-a mycorrhizal phytoremediation approach. Chemosphere 258:127337. doi: 10.1016/j.chemosphere.2020.127337
Kumar, A., Maiti, S. K., Tripti, Prasad, M. N. V., and Singh, R. S. (2017). Grasses and legumes facilitate phytoremediation of metalliferous soils in the vicinity of an abandoned chromite–asbestos mine. J. Soils Sediments 17, 1358–1368. doi: 10.1007/s11368-015-1323-z
Kumar, V., Pandita, S., Sidhu, G. P. S., Sharma, A., Khanna, K., Kaur, P., et al. (2021). Copper bioavailability, uptake, toxicity and tolerance in plants: a comprehensive review. Chemosphere 262:127810. doi: 10.1016/j.chemosphere.2020.127810
Kumpiene, J., Lagerkvist, A., and Maurice, C. (2008). Stabilization of As, Cr, Cu, Pb and Zn in soil using amendments: a review. Waste Manage. 28, 215–225. doi: 10.1016/j.wasman.2006.12.012
Kushwaha, A., Rani, R., Kumar, S., and Gautam, A. (2016). Heavy metal detoxification and tolerance mechanisms in plants: implications for phytoremediation. Environ. Rev. 24, 39–51. doi: 10.1139/er-2015-0010
Lago-Vila, M., Arenas-Lago, D., Rodríguez-Seijo, A., Andrade Couce, M. L., and Veja, F. A. (2015). Cobalt, chromium and nickel contents in soils and plants from a serpentinite quarry. Solid Earth 6, 323–335. doi: 10.5194/se-6-323-2015
Lewandowski, I., Scurlock, J. M. O., Lindvall, E., and Christou, M. (2003). The development and current status of perennial rhizomatous grasses as energy crops in the US and Europe. Biomass Bioenerg. 25, 335–361. doi: 10.1016/S0961-9534(03)00030-8
Li, C., Xiao, B., Wang, Q. H., Yao, S. H., and Wu, J. Y. (2014). Phytoremediation of Zn- and Cr-contaminated soil using two promising energy grasses. Water Air Soil Pollut. 225:2027. doi: 10.1007/s11270-014-2027-5
Li, T., Yang, X., Meng, F., and Lu, L. (2007). Zinc adsorption and desorption characteristics in root cell wall involving zinc hyperaccumulation in Sedum alfredii Hance. J. Zhejiang Univ. -Sci. B 8, 111–115. doi: 10.1631/jzus.2007.B0111
Lima, F. R. D., Barbosa, A. P., Vasques, I. C. F., Oliveira, J. R., Silva, A. O., Martins, G. C., et al. (2021). Physiological effects of mercury-contaminated Oxisols on common bean and oat. Environ. Sci. Pollut. Res. 28, 11279–11288. doi: 10.1007/s11356-020-11286-x
Lin, Y. F., and Aarts, M. G. M. (2012). The molecular mechanism of zinc and cadmium stress response in plants. Cell. Mol. Life Sci. 69, 3187–3206. doi: 10.1007/s00018-012-1089-z
Liu, R., Shan, C., Gao, Y., Wang, J., Xu, Z., Zhang, L., et al. (2016). Cerium improves the copper tolerance of turf grass Poa pratensis by affecting the regeneration and biosynthesis of ascorbate and glutathione in leaves. Braz. J. Bot. 39, 779–785. doi: 10.1007/s40415-015-0246-7
Liu, X., Shen, Y., Lou, L., Ding, C., and Cai, Q. (2009). Copper tolerance of the biomass crops Elephant grass (Pennisetum purpureum Schumach), Vetiver grass (Vetiveria zizanioides) and the upland reed (Phragmites australis) in soil culture. Biotechnol. Adv. 27, 633–640. doi: 10.1016/j.biotechadv.2009.04.017
Liu, Y., Wang, K., Xu, P., and Wang, Z. (2012). Physiological responses and tolerance threshold to cadmium contamination in Eremochloa ophiuroides. Int. J. Phytoremediat. 14, 467–480. doi: 10.1080/15226514.2011.587850
Loix, C., Huybrechts, M., Vangronsveld, J., Gielen, M., Keunen, E., and Cuypers, A. (2017). Reciprocal interactions between cadmium-induced cell wall responses and oxidative stress in plants. Front. Plant Sci. 8:1867. doi: 10.3389/fpls.2017.01867
Lomonte, C., Wang, Y., Doronila, A., Gregory, D., Baker, A. J. M., Siegele, R., et al. (2014). Study of the spatial distribution of mercury in roots of Vetiver grass (Chrysopogon zizanioides) by micro-pixe spectrometry. Int. J. Phytoremediat. 16, 1170–1182. doi: 10.1080/15226514.2013.821453
Lux, A., Martinka, M., Vaculík, M., and White, P. J. (2011). Root responses to cadmium in the rhizosphere: a review. J. Exp. Bot. 62, 21–37. doi: 10.1093/jxb/erq281
Ma, J. F., Yamaji, N., Mitani, N., Xu, X.-Y., Su, Y.-H., McGrath, S. P., et al. (2008). Transporters of arsenite in rice and their role in arsenic accumulation in rice grain. Proc. Natl. Acad. Sci. USA 105, 9931–9935. doi: 10.1073/pnas.0802361105
Mahbub, K. R., Kader, M., Krishnan, K., Labbate, M., Naidu, R., and Megharaj, M. (2017). Toxicity of inorganic mercury to native Australian grass grown in three different soils. Bull. Environ. Contam. Toxicol. 98, 850–855. doi: 10.1007/s00128-017-2096-4
Mahohi, A., and Raiesi, F. (2021). The performance of mycorrhizae, rhizobacteria, and earthworms to improve Bermuda grass (Cynodon dactylon) growth and Pb uptake in a Pb-contaminated soil. Environ. Sci. Pollut. Res. 28, 3019–3034. doi: 10.1007/s11356-020-10636-z
Malagoli, M., Rossignolo, V., Salvalaggio, N., and Schiavon, M. (2014). Potential for phytoextraction of copper by Sinapis alba and Festuca rubra cv. Merlin grown hydroponically and in vineyard soils. Environ. Sci. Pollut. Res. 21, 3294–3303. doi: 10.1007/s11356-013-2307-4
Malinowski, D. P., Zuo, H., Belesky, D. P., and Alloush, G. A. (2004). Evidence for copper binding by extracellular root exudates of tall fescue but not perennial ryegrass infected with Neotyphodium spp. endophytes. Plant Soil 267, 1–12. doi: 10.1007/s11104-005-2575-y
Marzban, L., Akhzari, D., Ariapour, A., Mohammadparast, B., and Pessarakli, M. (2017). Effects of cadmium stress on seedlings of various rangeland plant species (Avena fatua L., Lathyrus sativus L., and Lolium temulentum L.): growth, physiological traits, and cadmium accumulation. J. Plant Nutr. 40, 2127–2137. doi: 10.1080/01904167.2016.1269347
Mateos-Naranjo, E., Gallé, A., Florez-Sarasa, I., Perdomo, J. A., Galmés, J., Ribas-Carbó, M., et al. (2015). Assessment of the role of silicon in the Cu-tolerance of the C4 grass Spartina densiflora. J. Plant Physiol. 178, 74–83. doi: 10.1016/j.jplph.2015.03.001
Mbanga, O., Ncube, S., Tutu, H., Chimuka, L., and Cukrowska, E. (2019). Mercury accumulation and biotransportation in wetland biota affected by gold mining. Environ. Monit. Assess. 191:186. doi: 10.1007/s10661-019-7329-z
McGrath, S. P., and Zhao, F.-J. (2003). Phytoextraction of metals and metalloids from contaminated soils. Curr. Opin. Biotechnol. 14, 277–282. doi: 10.1016/S0958-1669(03)00060-0
Melato, F. A., Regnier, T., McCrindle, R. I., and Mokgalaka, N. S. (2012). Impact of metals on secondary metabolites production and plant morphology in vetiver grass (Chrysopogon zizanioides). S. Afr. J. Chem. 65, 178–183.
Mendoza-Cózatl, D. G., Butko, E., Springer, F., Torpey, J. W., Komives, E. A., Kehr, J., et al. (2008). Identification of high levels of phytochelatins glutathione and cadmium in the phloem sap of Brassica napus. A role for thiol-peptides in the long distance transport of cadmium and the effect of cadmium on iron translocation. Plant J. 54, 249–259. doi: 10.1111/j.1365-313X.2008.03410.x
Minerals Council of Australia [MCA] (2016). Mine Rehabilitation in the Australian Minerals Industry. Rehabilitation of Mining and Resources Projects as it Relates to Commonwealth Responsibilities. Canberra, Australia. (Industry Report, Submission 50). Available online at: https://www.minerals.org.au/sites/default/files/MCA_submission_to_the_Senate_mine_rehabilitation_inquiry_28_Apr_2017.pdf (accessed May 1, 2021).
Mishra, T., Pandey, V. C., Praveen, A., Singh, N. B., Singh, N., and Singh, D. P. (2020). Phytoremediation ability of naturally growing plant species on the electroplating wastewater-contaminated site. Environ. Geochem. Health 42, 4101–4111. doi: 10.1007/s10653-020-00529-y
Monnet, F., Vaillant, N., Hitmi, A., and Sallanon, H. (2005). Photosynthetic activity of Lolium perenne as a function of endophyte status and zinc nutrition. Funct. Plant Biol. 32, 131–139. doi: 10.1071/FP04129
Mu, J., Hu, Z., Xie, Z., Huang, L., and Holm, P. E. (2019). Influence of CaO-activated silicon-based slag amendment on the growth and heavy metal uptake of vetiver grass (Vetiveria zizanioides) grown in multi-metal-contaminated soils. Environ. Sci. Pollut. Res. 26, 32243–32254. doi: 10.1007/s11356-019-06429-8
Nabais, C., Labuto, G., Gonçalves, S., Buscardo, E., Semensatto, D., Nogueira, A. R. A., et al. (2011). Effect of root age on the allocation of metals, amino acids and sugars in different cell fractions of the perennial grass Paspalum notatum (bahiagrass). Plant Physiol. Biochem. 49, 1442–1447. doi: 10.1016/j.plaphy.2011.09.010
Nardis, B. O., Silva, E. B., Grazziotti, P. H., Alleoni, L. R. F., Melo, L. C. A., and Farnezi, M. M. M. (2018). Availability and zinc accumulation in forage grasses grown in contaminated soil. Int. J. Phytoremediat. 20, 205–213. doi: 10.1080/15226514.2017.1365347
Nazir, A., Malik, R. N., Ajaib, M., Khan, N., and Siddiqui, M. F. (2011). Hyperaccumulators of heavy metals of industrial areas of Islamabad and Rawalpindi. Pak. J. Bot. 43, 1925–1933.
Nguyen, T. Q., Hayward, A. R., Bruce, K. E., Hutchinson, T. C., and Emery, R. J. N. (2018). Chelator production by Deschampsia cespitosa (L.) Beauv. in adaptive Ni/Cu hyper-tolerance derived from fields in the Sudbury region and lab assessment. Botany 96, 711–721. doi: 10.1139/cjb-2017-0211
Nishizawa, A., Yabuta, Y., and Shigeoka, S. (2008). Galactinol and raffinose constitute a novel function to protect plants from oxidative damage. Plant Physiol. 147, 1251–1263. doi: 10.1104/pp.108.122465
Niu, K., Zhang, R., Zhu, R., Wang, Y., Zhang, D., and Ma, H. (2021). Cadmium stress suppresses the tillering of perennial ryegrass and is associated with the transcriptional regulation of genes controlling axillary bud outgrowth. Ecotox. Environ. Safe. 212:112002. doi: 10.1016/j.ecoenv.2021.112002
Niu, Z., Zhang, X., Wang, S., Ci, Z., Kong, X., and Wang, Z. (2013). The linear accumulation of atmospheric mercury by vegetable and grass leaves: potential biomonitors for atmospheric mercury pollution. Environ. Sci. Pollut. Res. 20, 6337–6343. doi: 10.1007/s11356-013-1691-0
Odinga, C. A., Kumar, A., Mthembu, M. S., Bux, F., and Swalaha, F. M. (2019). Rhizofiltration system consisting of Phragmites australis and Kyllinga nemoralis: evaluation of efficient removal of metals and pathogenic microorganisms. Desalin. Water Treat. 169, 120–132.
Opoku, P., Gikunoo, E., Arthur, E. K., and Foli, G. (2020). Removal of selected heavy metals and metalloids from an artisanal gold mining site in Ghana using indigenous plant species. Cogent Environ. Sci. 6:1840863. doi: 10.1080/23311843.2020.1840863
Osmolovskaya, N., Dung, V. V., and Kuchaeva, L. (2018). The role of organic acids in heavy metal tolerance in plants. Bio. Comm. 63, 9–16. doi: 10.21638/spbu03.2018.103
Panuccio, M. R., Logoteta, B., Beone, G. M., Cagnin, M., and Cacco, G. (2012). Arsenic uptake and speciation and the effects of phosphate nutrition in hydroponically grown kikuyu grass (Pennisetum clandestinum Hochst). Environ. Sci. Pollut. Res. 19, 3046–3053. doi: 10.1007/s11356-012-0820-5
Patra, J., Lenka, M., and Panda, B. B. (1994). Tolerance and co-tolerance of the grass Chloris barbata Sw. to mercury, cadmium and zinc. New Phytol. 128, 165–171. doi: 10.1111/j.1469-8137.1994.tb03999.x
Patra, M., Bhowmik, N., Bandopadhyay, B., and Sharma, A. (2004). Comparison of mercury, lead and arsenic with respect to genotoxic effects on plant systems and the development of genetic tolerance. Environ. Exp. Bot. 52, 199–223. doi: 10.1016/j.envexpbot.2004.02.009
Pelloux, J., Rustérucci, C., and Mellerowicz, E. J. (2007). New insight into pectin methylesterase structure and function. Trends Plant Sci. 12, 267–277. doi: 10.1016/j.tplants.2007.04.001
Peng, K., Li, X., Luo, C., and Shen, Z. (2006). Vegetation composition and heavy metal uptake by wild plants at three contaminated sites in Xiangxi area, China. J. Environ. Sci. Health Part A-Toxic/Hazard. Subst. Environ. Eng. 41, 65–76. doi: 10.1080/10934520500298838
Peralta-Videa, J. R., Lopez, M. L., Narayan, M., Saupe, G., and Gardea-Torresdey, J. (2009). The biochemistry of environmental heavy metal uptake by plants: implications for the food chain. Int. J. Biochem. Cell Biol. 41, 1665–1677. doi: 10.1016/j.biocel.2009.03.005
Pham, V. J. T., and Kim, J. (2012). Cultivation of unculturable soil bacteria. Trends Biotechnol. 30, 475–484. doi: 10.1016/j.tibtech.2012.05.007
Pietrini, F., Iannelli, M. A., Pasqualini, S., and Massacci, A. (2003). Interaction of cadmium with glutathione and photosynthesis in developing leaves and chloroplasts of Phragmites australis (Cav.) Trin. ex Steudel. Plant Physiol. 133, 829–837. doi: 10.1104/pp.103.026518
Pilon-Smits, E. A. H., Quinn, C. F., Tapken, W., Malagoli, M., and Schiavon, M. (2009). Physiological functions of beneficial elements. Curr. Opin. Plant Biol. 12, 267–274. doi: 10.1016/j.pbi.2009.04.009
Pinto, A. P., Simões, I., and Mota, A. M. (2008). Cadmium impact on root exudates of sorghum and maize plants: a speciation study. J. Plant Nutr. 31, 1746–1755. doi: 10.1080/01904160802324829
Pires-Lira, M. F., Castro, E. M., Lira, J. M., Oliveira, C., Pereira, F. J., and Pereira, M. P. (2020). Potential of Panicum aquanticum Poir. (Poaceae) for the phytoremediation of aquatic environments contaminated by lead. Ecotox. Environ. Safe. 193:110336. doi: 10.1016/j.ecoenv.2020.110336
Qian, X., Wu, Y., Zhou, H., Xu, X., Xu, Z., Shang, L., et al. (2018). Total mercury and methylmercury accumulation in wild plants grown at wastelands composed of mine tailings: insights into potential candidates for phytoremediation. Environ. Pollut. 239, 757–767. doi: 10.1016/j.envpol.2018.04.105
Rabêlo, F. H. S., and Borgo, L. (2016). Changes caused by heavy metals in micronutrient content and antioxidant system of forage grasses used for phytoremediation: an overview. Cienc. Rural 46, 1368–1375. doi: 10.1590/0103-8478cr20151291
Rabêlo, F. H. S., Borgo, L., and Lavres, J. (2018a). “The use of forage grasses for the phytoremediation of heavy metals: plant tolerance mechanisms, classifications, and new prospects,” in Phytoremediation: Methods, Management and Assessment, ed. V. Matichenkov (New York, NY: Nova Science Publishers), 59–103.
Rabêlo, F. H. S., Fernie, A. R., Navazas, A., Borgo, L., Keunen, E., Silva, B. K. A., et al. (2018b). A glimpse into the effect of sulfur supply on metabolite profiling, glutathione and phytochelatins in Panicum maximum cv. Massai exposed to cadmium. Environ. Exp. Bot. 151, 76–88. doi: 10.1016/j.envexpbot.2018.04.003
Rabêlo, F. H. S., Lux, A., Rossi, M. L., Martinelli, A. P., Cuypers, A., and Lavres, J. (2018c). Adequate S supply reduces the damage of high Cd exposure in roots and increases N, S and Mn uptake by Massai grass grown in hydroponics. Environ. Exp. Bot. 148, 35–46. doi: 10.1016/j.envexpbot.2018.01.005
Rabêlo, F. H. S., Silva, B. K. A., Borgo, L., Keunen, E., Rossi, M. L., Borges, K. L. R., et al. (2018d). Enzymatic antioxidants - relevant or not to protect the photosynthetic system against cadmium-induced stress in Massai grass supplied with sulfur? Environ. Exp. Bot. 155, 702–717. doi: 10.1016/j.envexpbot.2018.08.020
Rabêlo, F. H. S., Borgo, L., Merloti, L. F., Pylro, V. S., Navarrete, A. A., Mano, R. H., et al. (2020a). Effects of winter and summer conditions on Cd fractionation and bioavailability, bacterial communities and Cd phytoextraction potential of Brachiaria decumbens and Panicum maximum grown in a tropical soil. Sci. Total Environ. 728:138885. doi: 10.1016/j.scitotenv.2020.138885
Rabêlo, F. H. S., Lavres, J., Ramires, T. G., and Alleoni, L. R. F. (2020b). Diagnosis and recommendation integrated system and nutritional balance index reveal Cd-induced nutritional disorders in Panicum maximum assayed for Cd phytoextraction. Bioremediat. J. 24, 265–282. doi: 10.1080/10889868.2020.1841727
Rabêlo, F. H. S., de Andrade Moral, R., and Lavres, J. (2019). Integrating biochemical, morpho-physiological, nutritional, and productive responses to Cd accumulation in Massai grass employed in phytoremediation. Water Air Soil Pollut. 230:110. doi: 10.1007/s11270-019-4167-0
Rabêlo, F. H. S., Gaziola, S. A., Rossi, M. L., Silveira, N. M., Wójcik, M., Bajguzf, A., et al. (2021). Unraveling the mechanisms controlling Cd accumulation and Cd-tolerance in Brachiaria decumbens and Panicum maximum under summer and winter weather conditions. Physiol. Plant. 173, 20–44. doi: 10.1111/ppl.13160
Rabêlo, F. H. S., Jordão, L. T., and Lavres, J. (2017c). A glimpse into the symplastic and apoplastic Cd uptake by Massai grass modulated by sulfur nutrition: plants well-nourished with S as a strategy for phytoextraction. Plant Physiol. Biochem. 121, 48–57. doi: 10.1016/j.plaphy.2017.10.018
Rabêlo, F. H. S., Azevedo, R. A., and Monteiro, F. A. (2017a). Proper supply of S increases GSH synthesis in the establishment and reduces tiller mortality during the regrowth of Tanzania guinea grass used for Cd phytoextraction. J. Soils Sediments 17, 1427–1436. doi: 10.1007/s11368-016-1429-y
Rabêlo, F. H. S., Azevedo, R. A., and Monteiro, F. A. (2017b). The proper supply of S increases amino acid synthesis and antioxidant enzyme activity in Tanzania guinea grass used for Cd phytoextraction. Water Air Soil Pollut. 228:394. doi: 10.1007/s11270-017-3563-6
Radziemska, M., Gusiatin, Z. M., and Bilgin, A. (2017). Potential of using immobilizing agents in aided phytostabilization on simulated contamination of soil with lead. Ecol. Eng. 102, 490–500. doi: 10.1016/j.ecoleng.2017.02.028
Ram, B. K., Han, Y., Yang, G., Ling, Q., and Dong, F. (2019). Effect of hexavalent chromium [Cr(VI)] on phytoremediation potential and biochemical response of hybrid Napier grass with and without EDTA application. Plants 8:515. doi: 10.3390/plants8110515
Rauser, W. E., and Winterhalder, E. K. (1985). Evaluation of copper, nickel, and zinc tolerances in four grass species. Can. J. Bot. 63, 58–63. doi: 10.1139/b85-009
Redondo-Gómez, S., Mateos-Naranjo, E., Vecino-Bueno, I., and Feldman, S. R. (2011). Accumulation and tolerance characteristics of chromium in a cordgrass Cr-hyperaccumulator, Spartina argentinensis. J. Hazard. Mater. 185, 862–869. doi: 10.1016/j.jhazmat.2010.09.101
Reed, M., Warner, B. G., and Glick, B. R. (2005). Plant growth-promoting bacteria facilitate the growth of the Common Reed Phragmites australis in the presence of copper or polycyclic aromatic hydrocarbons. Curr. Microbiol. 51, 425–429. doi: 10.1007/s00284-005-4584-8
Ribeiro, P. G., Martins, G. C., Moreira, C. G., Oliveira, C., Andrade, M. L. C., Sales, T. S., et al. (2020). Interactions of cadmium and zinc in high zinc tolerant native species Andropogon gayanus cultivated in hydroponics: growth endpoints, metal bioaccumulation, and ultrastructural analysis. Environ. Sci. Pollut. Res. 27, 45513–45526. doi: 10.1007/s11356-020-10183-7
Richards, S. L., Wilkins, K. A., Swarbreck, S. M., Anderson, A. A., Habib, N., Smith, A. G., et al. (2015). The hydroxyl radical in plants: from seed to seed. J. Exp. Bot. 66, 37–46. doi: 10.1093/jxb/eru398
Richau, K. H., Kozhevnikova, A. D., Seregin, I. V., Vooijs, R., Koevoets, P. L., Smith, J. A. C., et al. (2009). Chelation by histidine inhibits the vacuolar sequestration of nickel in roots of the hyperaccumulator Thlaspi caerulescens. New Phytol. 183, 106–116. doi: 10.1111/j.1469-8137.2009.02826.x
Robinson, B. H., Brooks, R. R., Howes, A. W., Kirkman, J. H., and Gregg, P. E. H. (1997). The potential of the high-biomass nickel hyperaccumulator Berkheya coddii for phytoremediation and phytomining. J. Geochem. Explor. 60, 115–126. doi: 10.1016/S0375-6742(97)00036-8
Roohi, R., Jafari, M., Jahantab, E., Aman, M. S., Moameri, M., and Zare, S. (2020). Application of artificial neural network model for the identification the effect of municipal waste compost and biochar on phytoremediation of contaminated soils. J. Geochem. Explor. 208:106399. doi: 10.1016/j.gexplo.2019.106399
Rout, G. R., and Das, P. (2003). Effect of metal toxicity on plant growth and metabolism: I. Zinc. Agronomie 23, 3–11.
Rusinowski, S., Krzyzak, J., Sitko, K., Kalaji, H. M., Jensen, E., and Pogrzeba, M. (2019). Cultivation of C4 perennial energy grasses on heavy metal contaminated arable land: impact on soil, biomass, and photosynthetic traits. Environ. Pollut. 250, 300–311. doi: 10.1016/j.envpol.2019.04.048
Salas-Moreno, M., and Marrugo-Negrete, J. (2020). Phytoremediation potential of Cd and Pb-contaminated soils by Paspalum fasciculatum Willd. ex Flüggé. Int. J. Phytoremediat. 22, 87–97. doi: 10.1080/15226514.2019.1644291
Salt, D. E., Smith, R. D., and Raskin, I. (1998). Phytoremediation. Annu. Rev. Plant Physiol. Mol. Biol. 49, 643–668. doi: 10.1146/annurev.arplant.49.1.643
Santos, F. S., Hernández-Allica, J., Becerril, J. M., Amaral-Sobrinho, N., Mazur, N., and Garbisu, C. (2006). Chelate-induced phytoextraction of metal polluted soils with Brachiaria decumbens. Chemosphere 65, 43–50. doi: 10.1016/j.chemosphere.2006.03.012
Sarwar, N., Saifullah, Malhi, S. S., Zia, M. H., Naeem, A., Bibi, S., et al. (2010). Role of mineral nutrition in minimizing cadmium accumulation by plants. J. Sci. Food. Agric. 90, 925–937. doi: 10.1002/jsfa.3916
Schaaf, G., Ludewig, U., Erenoglu, B. E., Mori, S., Kitahara, T., and Von Wirén, N. (2004). ZmYS1 functions as a proton-coupled symporter for phytosiderophore- and nicotianamine-chelated metals. J. Biol. Chem. 279, 9091–9096. doi: 10.1074/jbc.M311799200
Schat, H., Sharma, S. S., and Vooijs, R. (1997). Heavy metal-induced accumulation of free proline in a metal-tolerant and a non-tolerant ecotype of Silene vulgaris. Physiol. Plant. 101, 477–482. doi: 10.1111/j.1399-3054.1997.tb01026.x
Schulz, H., Härtling, S., and Tanneberg, H. (2008). The identification and quantification of arsenic-induced phytochelatins-comparison between plants with varying As sensitivities. Plant Soil 303, 275–287. doi: 10.1007/s11104-007-9507-y
Selvi, A., Rajasekar, A., Theerthagiri, J., Ananthaselvam, A., Sathishkumar, K., Madhavan, J., et al. (2019). Integrated remediation processes toward heavy metal removal/recovery from various environments - a review. Front. Environ. Sci. 7:66. doi: 10.3389/fenvs.2019.00066
Senila, M. (2014). Real and simulated bioavailability of lead in contaminated and uncontaminated soils. J. Environ. Health Sci. Engineer. 12:108. doi: 10.1186/2052-336X-12-108
Sessitsch, A., Kuffner, M., Kidd, P., Vangronsveld, J., Wenzel, W. W., Fallmann, K., et al. (2013). The role of plant-associated bacteria in the mobilization and phytoextraction of trace elements in contaminated soils. Soil Biol. Biochem. 60, 182–194. doi: 10.1016/j.soilbio.2013.01.012
Seth, C. S., Remans, T., Keunen, E., Jozefczak, M., Gielen, H., Opdenakker, K., et al. (2012). Phytoextraction of toxic metals: a central role for glutathione. Plant Cell Environ. 35, 334–346. doi: 10.1111/j.1365-3040.2011.02338.x
Shabani, L., Sabzalian, M. R., and Mostafavi Pour, S. (2016). Arbuscular mycorrhiza affects nickel translocation and expression of ABC transporter and metallothionein genes in Festuca arundinacea. Mycorrhiza 26, 67–76. doi: 10.1007/s00572-015-0647-2
Shahid, M., Dumat, C., Khalid, S., Schreck, E., Xiong, T., and Niazi, N. K. (2017). Foliar heavy metal uptake, toxicity and detoxification in plants: a comparison of foliar and root metal uptake. J. Hazard. Mater. 325, 36–58. doi: 10.1016/j.jhazmat.2016.11.063
Sharifi, Z., Sinegani, A. A. S., and Shariati, S. (2012). Potential of indigenous plant species for the phytoremediation of arsenic contaminated land in Kurdistan (Iran). Soil Sediment. Contam. 21, 557–573. doi: 10.1080/15320383.2012.678951
Sharma, S. S., and Dietz, K.-J. (2006). The significance of amino acids and amino acid-derived molecules in plant responses and adaptation to heavy metal stress. J. Exp. Bot. 57, 711–726. doi: 10.1093/jxb/erj073
Sharma, S. S., Dietz, K.-J., and Mimura, T. (2016). Vacuolar compartmentalization as indispensable component of heavy metal detoxification in plants. Plant Cell Environ. 39, 1112–1126. doi: 10.1111/pce.12706
Shutcha, M. N., Mubemba, M. M., Faucon, M.-P., Luhembwe, M. N., Visser, M., Colinet, G., et al. (2010). Phytostabilisation of copper-contaminated soil in Katanga: an experiment with three native grasses and two amendments. Int. J. Phytoremediat. 12, 616–632. doi: 10.1080/15226510903390411
Silva, E. B., Souza, F. V. P., Grazziotti, P. H., Alleoni, L. R. F., Nardis, B. O., and Ferreira, E. A. (2017). Growth of tropical grasses in Oxisol contaminated by nickel. Chil. J. Agric. Res. 77, 273–280. doi: 10.4067/S0718-58392017000300273
Sinegani, A. A. S., and Dastjerdi, F. S. (2009). The accumulation of Zinc and Nickel in Irankoh indigenous plant species on a contaminated land. Soil Sediment. Contam. 18, 525–534. doi: 10.1080/15320380902978953
Singh, S., Parihar, P., Singh, R., Singh, V. P., and Prasad, S. M. (2016). Heavy metal tolerance in plants: role of transcriptomics, proteomics, metabolomics, and ionomics. Front. Plant Sci. 6:1143. doi: 10.3389/fpls.2015.01143
Singh, S., Sounderajan, S., Kumar, K., and Fulzele, D. P. (2017). Investigation of arsenic accumulation and biochemical response of in vitro developed Vetiveria zizanioides plants. Ecotox. Environ. Safe. 145, 50–56. doi: 10.1016/j.ecoenv.2017.07.013
Sipos, G., Solti, A., Czech, V., Vashegyi, I., Tóth, B., Cseh, E., et al. (2013). Heavy metal accumulation and tolerance of energy grass (Elymus elongatus subsp. ponticus cv. Szarvasi-1) grown in hydroponic culture. Plant Physiol. Biochem. 68, 96–103. doi: 10.1016/j.plaphy.2013.04.006
Smirnoff, N., and Stewart, G. R. (1987). Nitrogen assimilation and zinc toxicity to zinc-tolerant and non-tolerant clones of Deschampsia cespitosa (L.) Beauv. New Phytol. 107, 671–680. doi: 10.1111/j.1469-8137.1987.tb00905.x
Soares, C. R. F. S., and Siqueira, J. O. (2008). Mycorrhiza and phosphate protection of tropical grass species against heavy metal toxicity in multi-contaminated soil. Biol. Fertil. Soils 44, 833–841. doi: 10.1007/s00374-007-0265-z
Soleimani, M., Hajabbasi, M. A., Afyuni, M., Charkhabi, A. H., and Shariatmadari, H. (2009). Bioaccumulation of nickel and lead by bermuda grass (Cynodon dactylon) and tall fescue (Festuca arundiacea) from two contaminated soils. Casp. J. Environ. Sci. 7, 59–70.
Souza Junior, J. C., Nogueirol, R. C., and Monteiro, F. A. (2019). Nitrate and ammonium proportion plays a key role in copper phytoextraction, improving the antioxidant defense in Tanzania guinea grass. Ecotox. Environ. Safe. 171, 823–832. doi: 10.1016/j.ecoenv.2019.01.013
Steliga, T., and Kluk, D. (2020). Application of Festuca arundinacea in phytoremediation of soils contaminated with Pb, Ni, Cd and petroleum hydrocarbons. Ecotox. Environ. Safe. 194:110409. doi: 10.1016/j.ecoenv.2020.110409
Stypczyńska, Z., Dziamski, A., Jaworska, H., and Dąbkowska-Naskręt, H. (2018). Evaluation of the content of trace elements in the aerial and underground biomass of perennial grasses of the genus Miscanthus. Environ. Prot. Eng. 44, 141–151. doi: 10.37190/epe180310
Suszcynsky, E. M., and Shann, J. R. (1995). Phytotoxicity and accumulation of mercury in tobacco subjected to different exposure routes. Environ. Toxicol. Chem. 14, 61–67. doi: 10.1002/etc.5620140108
Taghizadeh, L. M., Kafi, M., and Moghadam, M. R. F. (2015). Breeding by in vitro culture to improve tolerance and accumulation of lead in Cynodon dactylon. J. Agr. Sci. Tech. 17, 1851–1860.
Tananonchai, A., and Sampanpanish, P. (2020). The influence of EDTA on the accumulation, movement, and distribution of cadmium in dwarf elephant grass. Environ. Technol. Innov. 17:100555. doi: 10.1016/j.eti.2019.100555
Tavili, A., Jahantab, E., Jafari, M., Motesharezadeh, B., Zargham, N., and Aman, M. S. (2019). Assessment of TPH and nickel contents associated with tolerant native plants in petroleum-polluted area of Gachsaran, Iran. Arab. J. Geosci. 12:325. doi: 10.1007/s12517-019-4478-x
Taylor, S. I., and Macnair, M. R. (2006). Within and between population variation for zinc and nickel accumulation in two species of Thlaspi (Brassicaceae). New Phytol. 169, 505–514. doi: 10.1111/j.1469-8137.2005.01625.x
Teodoro, M., Hejcman, M., Vítková, M., Wu, S., and Komárek, M. (2020). Seasonal fluctuations of Zn, Pb, As and Cd contents in the biomass of selected grass species growing on contaminated soils: implications for in situ phytostabilization. Sci. Total Environ. 703:134710. doi: 10.1016/j.scitotenv.2019.134710
Tiwari, S., Sarangi, B. K., Seralanathan, M. V., Sivanesan, S., Yadav, D., and Thul, S. T. (2015). Determination of arsenic extraction by Vetiveria zizanioides (L.) Nash plant for phytoremediation application. Chem. Ecol. 32, 1–11. doi: 10.1080/02757540.2015.1109080
Tordoff, G. M., Baker, A. J. M., and Willis, A. J. (2000). Current approaches to the revegetation and reclamation of metalliferous mine wastes. Chemosphere 41, 219–228. doi: 10.1016/S0045-6535(99)00414-2
Truyens, S., Jambon, I., Croes, S., Janssen, J., Weyens, N., Mench, M., et al. (2014). The effect of long-term Cd and Ni exposure on seed endophytes of Agrostis capillaris and their potential application in phytoremediation of metal-contaminated soils. Int. J. Phytoremediat. 16, 7–8. doi: 10.1080/15226514.2013.837027
Turnau, K., Ostachowicz, B., Wojtczak, G., Anielska, T., and Sobczyk, Ł (2010). Metal uptake by xerothermic plants introduced into Zn-Pb industrial wastes. Plant Soil 337, 299–311. doi: 10.1007/s11104-010-0527-7
Ukoh, S. N. B., Akinola, M. O., and Njoku, K. L. (2019). Comparative study on the remediation potential of Panicum maximum and Axonopus compressus in zinc (Zn) contaminated soil. Pollution 5, 687–699. doi: 10.22059/POLL.2019.272215.557
Ullah, S., Ali, R., Mahmood, S., Riaz, M. A., and Akhtar, K. (2020a). Differential growth and metal accumulation response of Brachiaria mutica and Leptochloa fusca on cadmium and lead contaminated soil. Soil Sediment. Contam. 29, 844–859. doi: 10.1080/15320383.2020.1777935
Ullah, S., Iqbal, Z., Mahmood, S., Akhtar, K., and Ali, R. (2020b). Phytoextraction potential of different grasses for the uptake of cadmium and lead from industrial wastewater. Soil Environ. 39, 77–86. doi: 10.25252/SE/2020/91796
Ullah, S., Mahmood, S., Ali, R., Khan, M. R., Akhtar, K., and Depar, N. (2021). Comparing chromium phyto-assessment in Brachiaria mutica and Leptochloa fusca growing on chromium polluted soil. Chemosphere 269:128728. doi: 10.1016/j.chemosphere.2020.128728
Ullah, S., Mahmood, T., Iqbal, Z., Naeem, A., Ali, R., and Mahmood, S. (2019). Phytoremediative potential of salt-tolerant grass species for cadmium and lead under contaminated nutrient solution. Int. J. Phytoremediat. 21, 1012–1018. doi: 10.1080/15226514.2019.1594683
van der Ent, A., Baker, A. J. M., Reeves, R. D., Pollard, A. J., and Schat, H. (2013). Hyperaccumulators of metal and metalloid trace elements: facts and fiction. Plant Soil 362, 319–334. doi: 10.1007/s11104-012-1287-3
van der Pas, L., and Ingle, R. A. (2019). Towards an understanding of the molecular basis of nickel hyperaccumulation in plants. Plants 8:11. doi: 10.3390/plants8010011
Vangronsveld, J., Colpaert, J., and Van Tichelen, K. (1996). Reclamation of a bare industrial area contaminated by non-ferrous metals: physico-chemical and biological evaluation of the durability of soil treatment and revegetation. Environ. Pollut. 94, 131–140. doi: 10.1016/S0269-7491(96)00082-6
Vangronsveld, J., Herzig, R., Weyens, N., Boulet, J., Adriaensen, K., Ruttens, A., et al. (2009). Phytoremediation of contaminated soils and groundwater: lessons from the field. Environ. Sci. Pollut. Res. 16, 765–794. doi: 10.1007/s11356-009-0213-6
Vangronsveld, J., Van Assche, F., and Clijsters, H. (1995). Reclamation of a bare industrial area, contaminated by non-ferrous metals: in situ metal immobilization and revegetation. Environ. Pollut. 87, 51–59. doi: 10.1016/S0269-7491(99)80007-4
Vargas, C., Pérez-Esteban, J., Escolástico, C., Masaguer, A., and Moliner, A. (2016). Phytoremediation of Cu and Zn by vetiver grass in mine soils amended with humic acids. Environ. Sci. Pollut. Res. 23, 13521–13530. doi: 10.1007/s11356-016-6430-x
Vázquez de Aldana, B. R., Gundel, P. E., García Criado, B., García Ciudad, A., García Sanchez, A., and Zabalgogeazcoa, I. (2014). Germination response of endophytic Festuca rubra seeds in the presence of arsenic. Grass Forage Sci. 69, 462–469. doi: 10.1111/gfs.12049
Vieira Filho, L. O., and Monteiro, F. A. (2020). Silicon modulates copper absorption and increases yield of Tanzania guinea grass under copper toxicity. Environ. Sci. Pollut. Res. 27, 31221–31232. doi: 10.1007/s11356-020-09337-4
Visconti, D., Álvarez-Robles, M. J., Fiorentino, N., Fagnano, M., and Clemente, R. (2020). Use of Brassica juncea and Dactylis glomerata for the phytostabilization of mine soils amended with compost or biochar. Chemosphere 260:127661. doi: 10.1016/j.chemosphere.2020.127661
Vymazal, J., and Březinová, T. (2016). Accumulation of heavy metals in aboveground biomass of Phragmites australis in horizontal flow constructed wetlands for wastewater treatment: a review. Chem. Eng. J. 290, 232–242. doi: 10.1016/j.cej.2015.12.108
Wang, J., Xiong, Y., Zhang, J., Lu, X., and Wei, G. (2020). Naturally selected dominant weeds as heavy metal accumulators and excluders assisted by rhizosphere bacteria in a mining area. Chemosphere 243:125365. doi: 10.1016/j.chemosphere.2019.125365
Wang, Y. D., and Greger, M. (2004). Clonal differences in mercury tolerance, accumulation, and distribution in willow. J. Environ. Qual. 33, 1779–1785. doi: 10.2134/jeq2004.1779
Wang, Z.-Y., and Ge, Y. (2006). Recent advances in genetic transformation of forage and turf grasses. In Vitro Cell. Dev. Biol.-Plant 42, 1–18. doi: 10.1079/IVP2005726
Weiersbye, I. M., Straker, C. J., and Przybylowicz, W. J. (1999). Micro-PIXE mapping of elemental distribution in arbuscular mycorrhizal roots of the grass, Cynodon dactylon, from gold and uranium mine tailings. Nucl. Instrum. Methods Phys. Res. Sect. B-Beam Interact. Mater. Atoms 158, 335–343. doi: 10.1016/S0168-583X(99)00467-X
Wen, W., Zhao, H., Ma, J., Li, Z., Li, H., Zhu, X., et al. (2018). Effects of mutual intercropping on Pb and Zn accumulation of accumulator plants Rumex nepalensis, Lolium perenne and Trifolium repens. Chem. Ecol. 34, 259–271. doi: 10.1080/02757540.2018.1427229
Wiangkham, N., and Prapagdee, B. (2018). Potential of Napier grass with cadmium-resistant bacterial inoculation on cadmium phytoremediation and its possibility to use as biomass fuel. Chemosphere 201, 511–518. doi: 10.1016/j.chemosphere.2018.03.039
Windham, L., Weis, J. S., and Weis, P. (2001). Patterns and processes of mercury release from leaves of two dominant salt marsh macrophytes, Phragmites australis and Spartina alterniflora. Estuaries 24, 787–795. doi: 10.2307/1353170
Wiszniewska, A., Hanus-Fajerska, E., Muszynská, E., and Ciarkowska, K. (2016). Natural organic amendments for improved phytoremediation of polluted soils: a review of recent progress. Pedosphere 26, 1–12. doi: 10.1016/S1002-0160(15)60017-0
Wong, C. K. E., and Cobbett, C. S. (2009). HMA P-type ATPases are the major mechanism for root-to-shoot Cd translocation in Arabidopsis thaliana. New Phytol. 181, 71–78. doi: 10.1111/j.1469-8137.2008.02638.x
Wu, B., Wang, Z., Zhao, Y., Gu, Y., Wang, Y., Yu, J., et al. (2019). The performance of biochar-microbe multiple biochemical material on bioremediation and soil micro-ecology in the cadmium aged soil. Sci. Total Environ. 686, 719–728. doi: 10.1016/j.scitotenv.2019.06.041
Xie, Y., Hu, L., Du, Z., Sun, X., Amombo, E., Fan, J., et al. (2014). Effects of cadmium exposure on growth and metabolic profile of Bermudagrass [Cynodon dactylon (L.) Pers.]. PLoS One 9:e115279. doi: 10.1371/journal.pone.0115279
Yadav, S. K. (2010). Heavy metals toxicity in plants: an overview on the role of glutathione and phytochelatins in heavy metal stress tolerance of plants. S. Afr. J. Bot. 76, 167–179. doi: 10.1016/j.sajb.2009.10.007
Yamaji, N., and Ma, J. F. (2014). The node, a hub for mineral nutrient distribution in graminaceous plants. Trends Plant Sci. 19, 556–563. doi: 10.1016/j.tplants.2014.05.007
Yang, S., Liang, S., Yi, L., Xu, B., Cao, J., Guo, Y., et al. (2014). Heavy metal accumulation and phytostabilization potential of dominant plant species growing on manganese mine tailings. Front. Environ. Sci. Eng. 8, 394–404. doi: 10.1007/s11783-013-0602-4
Yang, X. E., Baligar, V. C., Foster, J. C., and Martens, D. C. (1997). Accumulation and transport of nickel in relation to organic acids in ryegrass and maize grown with different nickel levels. Plant Soil 196, 271–276. doi: 10.1023/A:1004270528532
Yao, X., Niu, Y., Li, Y., Zou, D., Ding, X., and Bian, H. (2018). Heavy metal bioaccumulation by Miscanthus sacchariflorus and its potential for removing metals from the Dongting Lake wetlands, China. Environ. Sci. Pollut. Res. 25, 20003–20011. doi: 10.1007/s11356-018-2174-0
Yu, H., Yan, X., Zheng, X., Xu, K., Zhong, Q., Yang, T., et al. (2020). Differential distribution of and similar biochemical responses to different species of arsenic and antimony in Vetiveria zizanioides. Environ. Geochem. Health 42, 3995–4010. doi: 10.1007/s10653-020-00658-4
Yusuf, M., Fariduddin, Q., Hayat, S., and Ahmad, A. (2011). Nickel: an overview of uptake, essentiality and toxicity in plants. Bull. Environ. Contam. Toxicol. 86, 1–17. doi: 10.1007/s00128-010-0171-1
Zeven, A. C., and de Wet, J. M. J. (1982). Dictionary of Cultivated Plants and their Regions of Diversity, 2nd Edn. Wageningen: Centre for Agricultural Publishing and Documentation.
Zgorelec, Z., Bilandzija, N., Knez, K., Galic, M., and Zuzul, S. (2020). Cadmium and Mercury phytostabilization from soil using Miscanthus × giganteus. Sci. Rep. 10:6685. doi: 10.1038/s41598-020-63488-5
Zhang, J., Yang, N., Geng, Y., Zhou, J., and Lei, J. (2019). Effects of the combined pollution of cadmium, lead and zinc on the phytoextraction efficiency of ryegrass (Lolium perenne L.). RSC Adv. 9, 20603–20611. doi: 10.1039/C9RA01986C
Zhang, Y., Li, F., Xu, W., Ren, J., Chen, S., Shen, K., et al. (2019). Enhanced phytoextraction for co-contaminated soil with Cd and Pb by Ryegrass (Lolium perenne L.). Bull. Environ. Contam. Toxicol. 103, 147–154. doi: 10.1007/s00128-019-02661-7
Zhang, X., Gao, B., and Xia, H. (2014). Effect of cadmium on growth, photosynthesis, mineral nutrition and metal accumulation of bana grass and vetiver grass. Ecotox. Environ. Safe. 106, 102–108. doi: 10.1016/j.ecoenv.2014.04.025
Zhang, X., Zhang, X., and Huang, K. (2016). Phytostabilization of acidic soils with heavy metal contamination using three forage grasses in combination with organic and inorganic amendments. Soil Sediment. Contam. 25, 459–475. doi: 10.1080/15320383.2016.1168357
Zhao, F.-J., Ma, J. F., Meharg, A. A., and McGrath, S. P. (2009). Arsenic uptake and metabolism in plants. New Phytol. 181, 777–794. doi: 10.1111/j.1469-8137.2008.02716.x
Zhao, A., Gao, L., Chen, B., and Feng, L. (2019). Phytoremediation potential of Miscanthus sinensis for mercury-polluted sites and its impacts on soil microbial community. Environ. Sci. Pollut. Res. 26, 34818–34829. doi: 10.1007/s11356-019-06563-3
Zhao, J., Xia, B., Meng, Y., Yang, Z., Pan, L., Zhou, M., et al. (2019). Transcriptome analysis to shed light on the molecular mechanisms of early responses to cadmium in roots and leaves of King Grass (Pennisetum americanum × P. purpureum). Int. J. Mol. Sci. 20:2532. doi: 10.3390/ijms20102532
Zheng, Q., Cheng, Z. Z., and Yang, Z. M. (2013). HISN3 mediates adaptive response of Chlamydomonas reinhardtii to excess nickel. Plant Cell Physiol. 54, 1951–1962. doi: 10.1093/pcp/pct130
Zhiguo, E., Tingting, L., Chen, C., and Lei, W. (2018). Genome-wide survey and expression analysis of P1B-ATPases in rice, maize and sorghum. Rice Sci. 25, 208–217. doi: 10.1016/j.rsci.2018.06.004
Zhou, Z., Guo, Y., Hu, L., He, L., Xu, B., Huang, Z., et al. (2020). Potential use of king grass (Pennisetum purpureum Schumach. × Pennisetum glaucum (L.) R.Br.) for phytoextraction of cadmium from fields. Environ. Sci. Pollut. Res. 27, 35249–35260. doi: 10.1007/s11356-020-09844-4
Zhu, H., Chen, L., Xing, W., Ran, S., Wei, Z., Amee, M., et al. (2020). Phytohormones-induced senescence efficiently promotes the transport of cadmium from roots into shoots of plants: a novel strategy for strengthening of phytoremediation. J. Hazard. Mater. 388:122080. doi: 10.1016/j.jhazmat.2020.122080
Keywords: heavy metals, phytoextraction, phytofiltration, phytostabilization, Poaceae, tolerance mechanisms, toxicity, trace elements uptake
Citation: Rabêlo FHS, Vangronsveld J, Baker AJM, van der Ent A and Alleoni LRF (2021) Are Grasses Really Useful for the Phytoremediation of Potentially Toxic Trace Elements? A Review. Front. Plant Sci. 12:778275. doi: 10.3389/fpls.2021.778275
Received: 16 September 2021; Accepted: 19 October 2021;
Published: 24 November 2021.
Edited by:
Raul Antonio Sperotto, Universidade do Vale do Taquari (UNIVATES), BrazilReviewed by:
Luisa Louro Martins, University of Lisbon, PortugalBhumi Nath Tripathi, Indira Gandhi National Tribal University, India
Copyright © 2021 Rabêlo, Vangronsveld, Baker, van der Ent and Alleoni. This is an open-access article distributed under the terms of the Creative Commons Attribution License (CC BY). The use, distribution or reproduction in other forums is permitted, provided the original author(s) and the copyright owner(s) are credited and that the original publication in this journal is cited, in accordance with accepted academic practice. No use, distribution or reproduction is permitted which does not comply with these terms.
*Correspondence: Flávio Henrique Silveira Rabêlo, flaviohsr.agro@usp.br